The heart of any vacuum furnace is said to be its hot zone and if properly constructed and well maintained will help ensure that the furnace performs in an optimal manner. One of the most important aspects of the hot zone is its insulation and the choice of materials used in its construction. In this article, we will focus on the two main Hot Zone configurations namely all-metal hot zones and carbon-based hot zones.
Thermal Insulating Systems
We begin, however, with a brief discussion of the three modes of heat transfer, namely convection, radiation, and conduction (Fig 1). To perform properly, the hot zone insulating system must contend with all three.
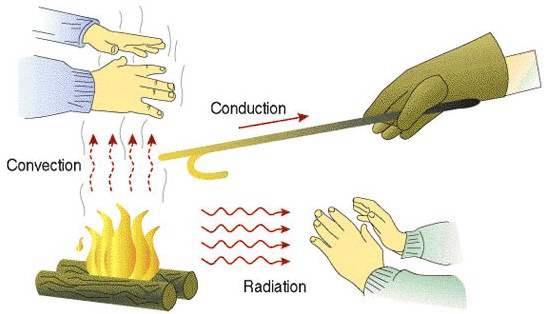
In simplest terms, conduction and radiation may be thought of transferring energy to the surface of the part, while conduction is responsible for the heat to penetrate into the center of the parts.
Conduction or conductive heat transfer occurs by direct molecular collision. On a microscopic scale, the kinetic energy of molecules varies in direct proportion to their temperature. So, as the temperature rises the molecules increase in motion and gain kinetic energy. They then collide with cooler surface molecules, transferring their kinetic energy and hence their heat, to the part surface (Fig. 2).
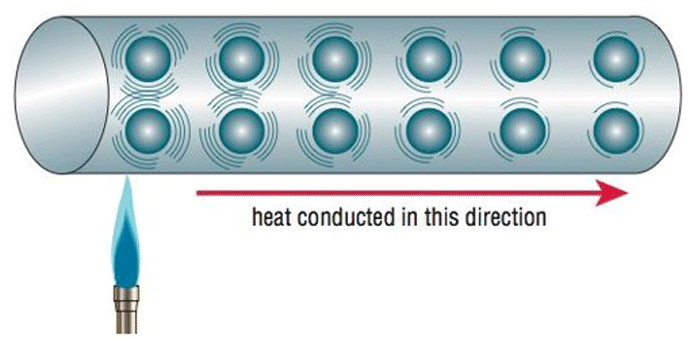
Since this happens only when there is direct physical contact between a hot and a cool material, conduction heat transfer is of particular concern in hot zone design wherever there are penetrations through the furnace wall, such as cooling nozzles, fittings, thermocouple ports, or structural hearth supports to name a few. These openings provide a direct path for the heat to conduct from the inside of the hot zone to the outside cage or walls of the furnace. For non-metallic insulation systems, the insulation (ceramic, graphite board or blanket) must be designed to minimize conduction, since this is the primary means by which heat will be transferred through the insulation.
Convection heat transfer in an insulation system occurs when a gas such as air carries the heat from the hot side of the insulating system to the cool side, penetrating through the insulation due to its porosity. Although the insulation in non-vacuum furnace styles must be designed with convection heat transfer in mind, vacuum furnaces are somewhat insensitive to convective heat transfer once the furnace is operating under vacuum. As long as the chamber is under vacuum during operation, this mode of heat transfer is practically non-existent, as convection requires the presence of gas molecules to transfer heat energy by bulk movement, and there are few molecules present in the evacuated chamber. Where convection plays a role is in convection heating where the furnace is backfilled with nitrogen or another inert gas to positive pressure (typically 1 – 2 bar) and a convection fan circulates the gas to preheat the load. Another instance where convection plays a role is when partial pressure is used, for example, to prevent vaporization of elements present in the parts (such as chromium in stainless steels). Convection also plays a significant role during high-pressure gas quenching.
The third mode of heat transfer, radiant transfer, occurs when the temperature difference between two bodies causes heat energy, in the form of electromagnetic waves, to travel from the hotter body to the cooler body. Since the rate of radiant heat transfer is proportional to the fourth power of the (absolute) temperature of the heat emitting surface, a considerable amount of heat energy can theoretically be transferred through the furnace walls by radiant means as the furnace temperature rises. In addition, radiant heat does not require a medium or intermediate material to transfer the heat. In fact, a vacuum is the optimal condition for transferring radiant heat since in a vacuum there is no gas in the way to scatter or absorb it. As a result, the insulating system in a vacuum furnace must be specifically designed to reduce radiant heat transfer.
There are several insulating system designs common in vacuum furnaces
- All metal hot zone design
- All graphite hot zone design
- Composite designs using both metal and graphite or ceramic insulation
All-Metal Construction
We now want to discuss how all-metal hot zone construction, consisting of layers of heat shields minimizes heat transfer through the furnace wall. Most standard vacuum furnaces operate at either a maximum temperature of 1315ºC (2400ºF) or 1650ºF (3000ºF). Some manufacturers have extended the lower temperature rating to 1340ºC (2450ºF) or 1450ºC (2650ºF) to accommodate high-temperature bake out cycles. Specialized vacuum furnaces are capable of operating at much higher temperatures, up to 3,000°C (5,400°F), and in these instances, the insulation system must be very carefully engineered due to the increased radiant energy effects.
Today, hot zones are primarily constructed in a so-called all-metal construction or all graphite construction. Composite hot zones are still being produced, the most common of which involved metallic elements and graphite insulation or graphite elements and metallic shields.
In an all-metal hot zone, the insulation system consists of multiple layers of thin metal with gaps between them (Fig. 3). These strips of metal are referred to as heat shields, the layers having an air gap (or perhaps more accurately a vacuum gap) between them to retard the transfer of radiant energy.
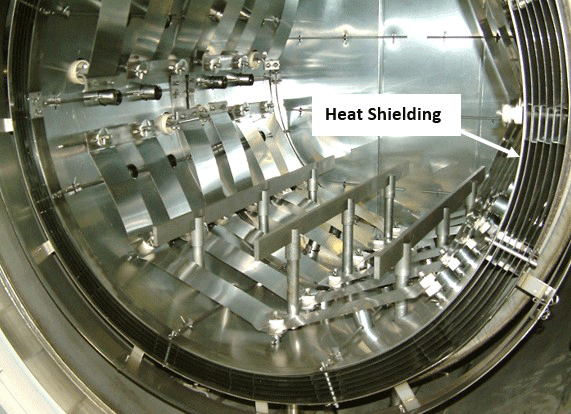
The number of heat shields and their materials of construction is a function of the temperature rating of the furnace. Each shield has an additive insulating effect. It is common for the system to incorporate a total of five or six shields, two or more layers of molybdenum on the hot face, backed by several layers of type 304 or 316 stainless steel. Molybdenum has a maximum operating temperature of 1700°C (3100°F), in comparison to 980°C (1800°F) for stainless steel. Molybdenum also has a superior (lower) emissivity than stainless steel (Table 1). This reduced emissivity allows it to reflect more of the radiant heat and absorb less.
Tantalum sheet, with a maximum operating temperature of 4350° F (2400° C), is used in place of molybdenum for higher temperature furnaces, as is tungsten. Tantalum also has a very favorable emissivity.
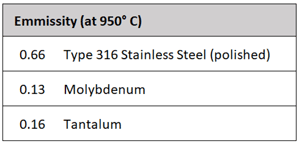
Molybdenum and molybdenum alloys experience a phase change at temperatures somewhere above 1150°C (2100°F) depending on the alloy and the result is that the molybdenum becomes brittle. Embrittled (aka recrystallized) heat shields will readily fracture along the grain boundaries when impact loaded and are susceptible to damage in an industrial setting so extreme care must be taken when inspecting or performing maintenance on an all-metal hot zone.
Recrystallization also limits the use of pure molybdenum for furnace racks and high-temperature fixtures. In order to reduce embrittlement, molybdenum can be doped with lanthanum oxide or other rare earth elements. This is in contrast to alloying, where a larger amount of the secondary material is combined with the primary to produce a distinct alloy. Lanthanum oxide doping stabilizes the molybdenum grain structure, reducing brittleness in the event of recrystallization. Lanthanum oxide holds the grain growth of the molybdenum grain boundaries to a tolerable level, at which strength and ductility are preserved. Whereas undoped molybdenum’s grain boundaries continue to grow and expand to the point of embrittlement, doped molybdenum resists embrittlement at temperatures up to 1850°C (3360°F).
Although all metal hot zones tend to be more expensive than their graphite counterparts due in large part to the high cost of molybdenum and/or tantalum, as well as the higher labor costs involved with their manufacture, they are appealing for ultra clean applications such as processing aerospace parts or medical devices, especially those involving titanium or superalloys.
The Importance of Emissivity
Since the function of the all-metal insulating system depends heavily on its ability to reflect radiant heat, it is worth taking a moment to review the property of emissivity, which quantifies this. Emissivity defines the proportion of radiant energy a material, such as a metal reflector, absorbs vs. how much it reflects. A material with an emissivity of 0 would absorb 0% of the radiant energy and reflect 100% of it. A material with an emissivity of 1.0 would reflect none of the heat, but rather would absorb all of it.
The goal, therefore, when designing all-metal insulating systems is to select materials with a low emissivity, and to maintain this low emissivity over time. One of the enemies of good reflector performance is oxidation. An oxidized metal surface has a greatly increased emissivity over a clean surface (Fig. 4), and therefore reflects much less of its heat energy. One other item worth noting is that emissivity of a material is also dependent on the wavelength of the radiant heat, which is itself dictated by its temperature. Higher temperatures correspond to shorter wavelengths. This is advantageous for vacuum furnaces because at temperatures above 695°C (1280°F), corresponding to a wavelength of 3 microns, the emissivity starts to reach a minimum, and therefore the maximum heat energy is reflected. At higher temperatures, the wavelength continues to drop slightly and then level off.
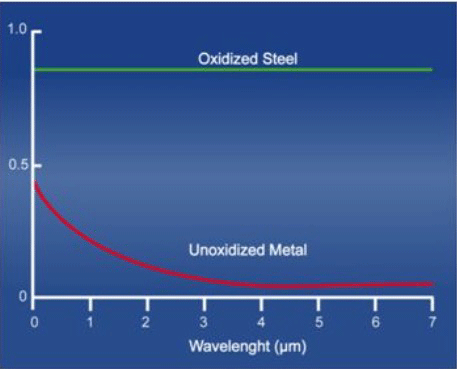
Contamination of All Metal Insulating Systems
The principal issue with all-metal insulating systems is the loss of emissivity due to contamination of the metal heat shields. This can be due to air leaking into the chamber, oxygen/water vapor present in the inert gas backfill lines, or to contaminated backfill gas. Backfill lines, regulators, valves, and other components undergo considerable operational stress during repeated quench and reheat cycles. Although the equipment is tight when brand new, after years of repeated cycling the seals and threaded connections start to leak, components begin to fail, and soft solder joints can experience cracking. This allows air to leak into the chamber and causes surface oxidation and dulling of the heat shields and furnace interior (Fig. 5).
The result of air infiltration is a reduction in the reflectiveness of the shields, and since they insulate by reflecting radiant heat, this dulling process drastically reduces the effectiveness of an all-metal insulating system. One of the ways to detect this (other than visually) is to monitor power usage, which will increase as the thermal efficiency of the hot zone deteriorates. At some point, the furnace, which may have originally only required 50 – 60% of the installed power, has a difficult time reaching temperature even at 90 – 100% input.
Over time, this gradual contamination results in less effective insulating of the furnace and increased power consumption of the furnace heating system. Not only does the furnace consume more power to make up for these losses, but the external cooling system that removes the heat from the shell in the cold wall design has to work harder to remove that heat. As a result, the owner pays for the loss of insulating efficiency not once, but twice.
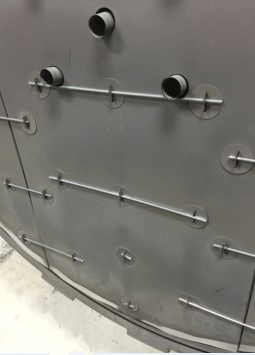
In addition to gradual contamination caused by air leaks, the heat shielding can be oxidized in the event of a mishap, such as opening the furnace door at elevated temperature or the failure of a component which allows air entry. Oxygen-laden air enters quickly and contaminates the heat shields. This can be severe enough to render the furnace unusable, despite attempts to run hydrogen clean-up cycles, forcing the shielding to be replaced.
Another way heat shielding can change emissivity is from the release of contaminants from the load, such as oils, greases, binders, brazing pastes and other outgassed products, which accumulate over time to render the shielding ineffective. To prevent this, care must be taken to properly clean the loads.
Still another source of heat shielding contamination is moisture from the factory during loading and unloading of the furnace, or if the furnace is turned off and the left open when not in operation. If the dew point of the air is below the temperature of the cold wall, condensation will occur, depositing moisture, which will release its oxygen under vacuum, and cause oxidation of the heat shielding.
Graphite hot zone construction.
As was discussed above, the heart of any vacuum furnace is its hot zone. If properly designed, constructed and maintained it will help ensure that your furnace performs in an optimal manner. One of the most important aspects of the hot zone is its insulation and the choice of materials used in its construction. The focus here will be on graphite-lined hot zones.
A popular alternative to all metal lined hot zones utilizes graphite-based insulation. A typical design for a maximum operating temperature of 1315ºC (2400ºF) consists of a 50 mm (2 in.) thick graphite material, typically in the form of (felt) blanket or board (Fig. 6). It is not uncommon to have two or more layers of material. For those familiar with high-temperature atmosphere furnaces, this insulation thickness may seem surprising considering the process temperatures inside the furnace. However, the thermal characteristics of graphite materials make it possible. In addition, the outer shell of the furnace is typically water-cooled and the fact that most processes run either under vacuum or in a partial pressure atmosphere further reduces heat transfer. If it were not for the cooling jacket, the exterior temperature could be in the range of 150 – 260°C (300 – 500° F).
The graphite blanket or board is usually installed in layers, and during installation, the layers are staggered to minimize the likelihood of continuous gaps from inside to outside and subsequent heat loss. A graphite felt-lined hot zone may consist of individual layers either 6 mm ( ¼”) or 12 mm (½”) thick. Graphite board is typically in 25 mm (1”) layers. Blanket products can subsequently be covered by a graphite paint, and board products can be supplied with a hot face covering of graphite foil or foil-bonded carbon composite. These improve reflectivity (i.e., reduced emissivity) of the heat from the graphite heaters back toward the load as well as protect the surface from damage by process or quench gases. There can also be intermediate layers of foil sandwiched between the layers of graphite (Fig. 7). These additional foil layers provide increased insulating efficiency. One test of four foil layers in between graphite boards showed an improvement of 10% to 35% as successive layers are added.7
In a graphite insulation system, the graphite blanket/board has a low thermal conductivity (Fig. 8), which resists heat transfer from the hot side to the cool side. With a thermal conductivity three times better (lower) than metal heat shielding, these systems provide superior insulating characteristics in comparison to all-metal hot zones and are normally less expensive and take less time to install. In comparing graphite blanket to graphite board, the blanket option is generally less expensive, as durable, and arguably easier to maintain.
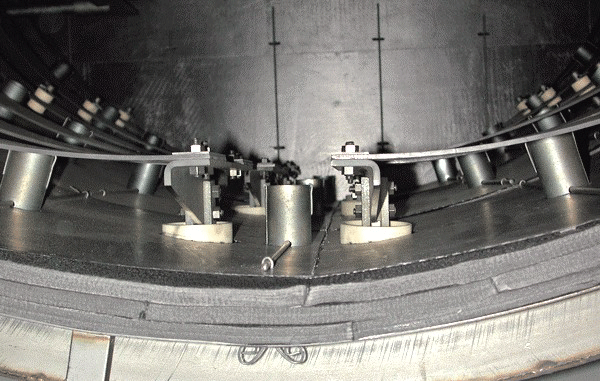
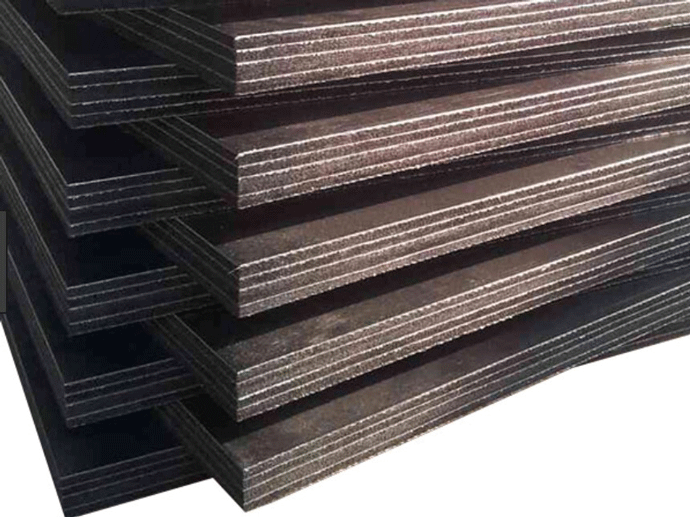
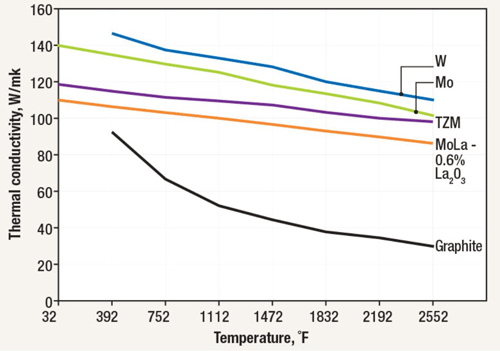
Graphite insulation comes in a variety of styles and configurations. The typical materials of construction include: graphite felt, rigid graphite board (coated, uncoated, and foil-bonded), graphite foil, carbon fiber reinforced carbon (CFC or C/C), and non-fibrous board (foil board). All of these products work by blocking the radiant heat emitted from the interior of the hot zone, which minimizes the heat transfer to the cage supporting the insulation and ultimately to the cold wall. Because of graphite’s low conductivity, these hot zones are typically more energy-efficient than all-metal designs.
One of the disadvantages of graphite materials is that they have a high specific heat (cp), a property that defines how much thermal energy a material can hold. Compared to molybdenum, a common metallic heat shielding, graphite’s specific heat is more than double (Table 1). Therefore, a given weight of graphite will retain twice the heat energy as molybdenum. This results in a slightly reduced quenching capability because some of the cooling capacity of the quenching gas is needed to bring down the insulation temperature (Fig. 9). In addition to the thermal mass of the insulation containing excess heat, graphite is also (by design) a poor conductor, so its heat is trapped within the graphite fibers, delaying the release of its heat energy during quenching. The multiple separated layers of heat shielding used in the all-metal insulation design, on the other hand, allow the quench gas to freely flow between them, allowing for more rapid quenching.
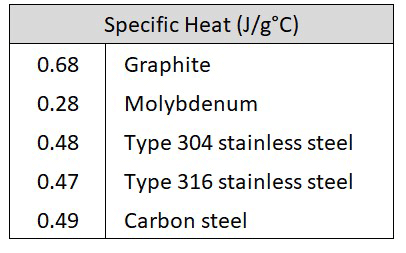
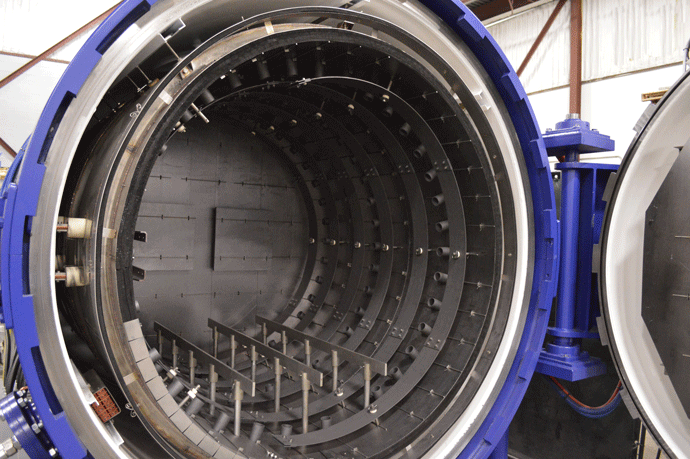
Graphite blanket (felt) is a rayon-based insulation supplied in rolls (Fig 10) and is convenient to work with as it is easily cut with scissors or a box cutter. It can be formed and sewn into various shapes using carbon cord as a thread. Common sizes are 1.067 – 1.200 mm (42” and 47”) wide, with thicknesses from 3 – 13 mm (0.12” – 0.50”), and densities of 0.0005 – 0.001 g/cc. Graphite felt is ideal for many vacuum furnace applications as it exhibits minimal shrinkage at temperature, has low thermal conductivity and a high sublimation temperature (i.e., the temperature at which it will evaporate into a gas), namely 3600°C (6512°F). For applications where chemical purity is not critical, carbon felt is used as a less expensive alternative to graphite felt. It has a maximum temperature rating, however of only 1000°C (1832°F).
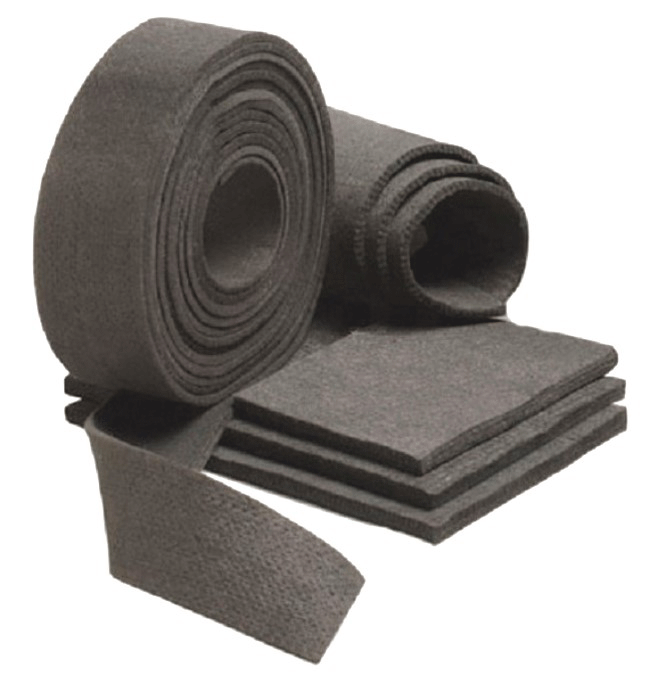
Graphite board (Fig. 11) is similar to graphite felt, except it is infused with carbon binders under compression and heated to form a rigid structure, in a process referred to as carbonizing and graphitizing. Graphite board is used at temperatures up to 2200°C (3992°F) and vacuum levels to 10-5 mbar (7.5 x 10-6 Torr). The board can be coated with graphite foil (aka foil-bonding) on one or both sides, to allow it to better reflect the radiant heat from the heating elements back into the hot zone, which improves its insulating effectiveness. The graphite foil also provides a barrier against gas penetration. Graphite boards can also be coated with a carbon composite fabric to provide extra mechanical stability and improved resistance to gas erosion, as experienced during high-pressure gas quenching for example. Sacrificial layers of carbon composite material or graphite-foil products are also used to cover the bottom third of a hot zone when performing brazing operations. Graphite boards can be molded into special shapes (Fig. 12) or machined for specific applications.
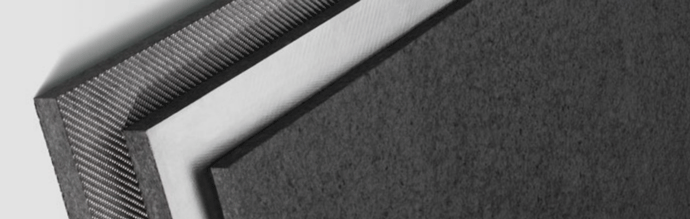
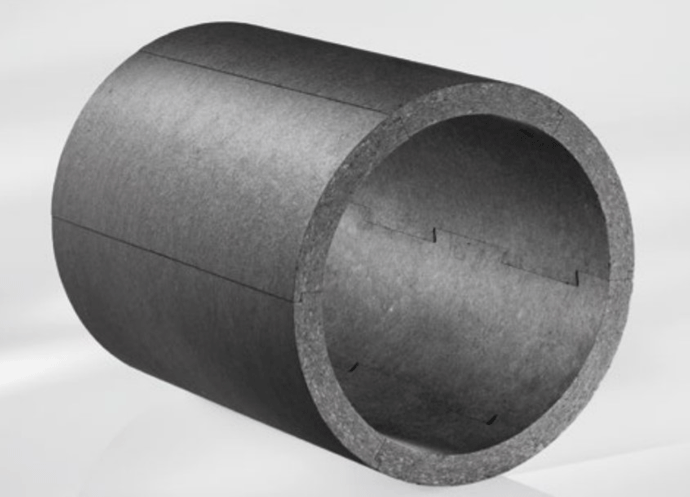
PAN vs Rayon Graphite Felt
Graphite felt is made from either of two source materials, polyacrylonitrile (PAN) or rayon, which are known as precursors. The first step in the process, carbonization, refers to the conversion of an organic substance into carbon material by heating it in the absence of air. Fossil fuels, for example, result from the carbonization of vegetation. After the precursors are carbonized, the resulting carbon is then heated to approximately 3000°C (5432°F) in the absence of oxygen. This causes the carbon crystallites to grow and rearrange in an orderly layered hexagonal structure of parallel planes (Fig. 13). This conversion is called graphitization, which causes a change in the physical properties of the material, giving it the desired strength and other properties.
The resulting graphite has a huge molecular structure with numerous, strong covalent bonds that require a lot of energy to separate the carbon atoms, giving graphite its temperature resistance properties. Graphitization also removes impurities from the material, and additional steps can produce graphite well over 99.9% pure. The high carbon content fibers resulting from graphitization are then used to manufacture flexible felt insulation, which can then be rigidized into boards.
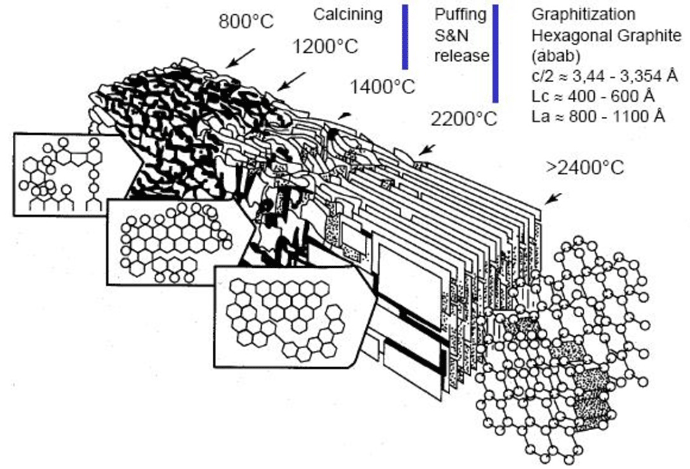
Although graphitization is used for making both PAN and rayon based graphite, graphite that uses rayon as the precursor is a higher-performing material than PAN, and has better insulating characteristics (Fig 14), particularly at higher temperatures. A 20% improvement in insulating efficiency has been documented with rayon in comparison to PAN7. However, rayon-based graphite is more expensive. One source advertised prices (in February 2018) of $30 per pound for PAN, and $44 per pound for rayon-based graphite.5
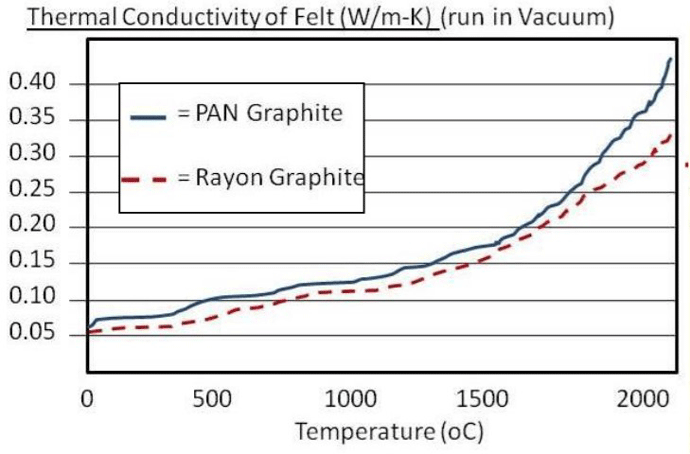
Gas Erosion and De-Binding
When considering graphite insulation one must be aware of the potential for erosion of the material due to abrasion from the quenching gas. This problem is most often observed in the pressure range of 6 – 20 bar along the exposed joints and penetrations through the boards when rapid gas quenching. For this reason, many of these areas are capped or covered by another material such as molybdenum or carbon composite. At a pressure of 20 bar, a quenching gas such as nitrogen has a weight of 51 kg/m3 (1.4 lb/ft3), so it’s easy to understand how high-velocity gas quenching can so easily erode the graphite board. In addition to the reduction in insulating efficiency due to loss of insulation thickness, the other problem resulting from gas erosion is the fine graphite powder that results. This powder will be carried by the quenching gas through the heat exchanger and blower of the gas quench system. It then collects inside the heat exchanger, decreasing its efficiency. In addition, the graphite powder can contaminate the work and interfere with the process being performed in the furnace.
Non-Fibrous Foam Board
In addition to erosion from the quenching gas, another source of fugitive graphite powder is vaporization of the binder from the board (aka de-binding). Without the binder, the graphite is liberated as a fine powder that can contaminate the process and clog the quench gas cooling system, in the same way, the abraded powder can.
An alternative to graphite felt is non-fibrous carbon foam board. This has received mixed reviews in the industry. Similar to graphite board, carbon foam offers high-temperature resistance up to roughly 2800°C (5072°F) and good resistance to thermal shock. Since it is not manufactured by adding binders to felt, there are no binders to evaporate out of the insulation, and de-binding is not a concern. Foam boards offer low thermal conductivity, high mechanical stability and a mostly closed cell design that impedes gas penetration. One drawback of this material in comparison to conventional carbon board is its lack of mechanical rigidity. It is fragile and breaks easily. It also has a higher thermal conductivity than rigidized graphite board, and only offers 25% of the thermal insulating capability. Therefore, it must be four times thicker to provide the same insulating quality.
Graphite Decomposition When Exposed to Oxygen
A major concern with the use of graphite insulation is the potential for it to decompose when exposed to air. If oxygen is present at temperatures above approximately 300°C (572ºF), the carbon that makes up the graphite will combine with oxygen to form CO and CO2 (Equation 1).
(1) C + ½O2 = CO and C + O2 = CO2
The result is that the graphite will slowly turn into CO and CO2 gas, which will be removed from the furnace by the pumping system. At first, pitting occurs (Fig. 15), often observed as a “sugar cube” surface appearance10 and as further decomposition ensues, the graphite continues to lose mass (Fig. 16) and simply disappears.
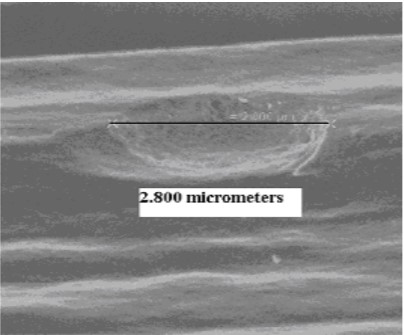
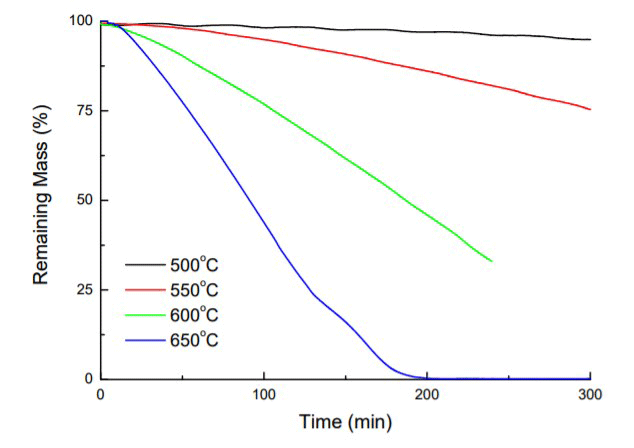
Summary
A majority of today’s vacuum heat-treating furnaces are supplied with graphite hot zones given the tremendous versatility of this material choice. Graphite insulation has improved significantly over the past several decades with respect to thermal stability and product integrity over a wide temperature and application range. Just as with all metal-lined vacuum furnaces, a leak-tight vacuum furnace system ensures that air (oxygen) and water vapor are kept out of the hot zone. Clean, dry work entering the furnace extends the overall life of all hot zone materials.
References
- CeraMaterials (www.ceramaterials.com)
- Vac-Aero International (www.vacaero.com)
- Smore.com
- Pakton.net
- Plansee (www.plansee.com)
- Ircon, A Fluke Company (www.fluke.com)
- VAC AERO International (https://vacaero.com)
- Industrial Heating (http://www.industrialheating.com)
- Graphite Store (http://www.graphitestore.com)
- SGL Group (http://www.sglgroup.com/cms/international/home/index.html?__locale=en)
- CeraMaterials (http://www.ceramaterials.com)
- Graphite Concept Products (http://www.graphiteconcept.com/component/option,com_frontpage/Itemid,1/)
- Herring, Daniel H., Vacuum Heat Treatment, BNP Media, 2012.
- Fradette, Real, “Methods of Improving Vacuum Furnace Insulation Efficiencies”, Industrial Heating, September 2013.
- Sinotek Materials (http://www.cn-materials.com)
- Herring, Daniel H., Vacuum Heat Treatment, Volume II, BNP Media, 2016.
- “Strength Degradation of Glass and Carbon Fibres at High Temperature”, S. Feih, E. Boiocchi, E. Kandare, Z. Mathys, A.G. Gibson and A.P. Mouritz