A frequently asked question is, “How can I keep my vacuum furnace performing like it was when it was brand new?” This goes hand in hand with the question “How should I operate and maintain my vacuum furnace to maximize my investment and produce repeatable high-quality results?” In this article will provide seven (7) important tips for doing just this.
Here are the Seven (7) Tips we will be Covering:
Tip #1: Maintaining Your Vacuum Pumps
Tip #2: Selecting the Right Vacuum Level for the Job
Tip #3: Avoiding Eutectic Melting
Tip #4: Maintaining Your “O” Rings Seals
Tip #5: Avoiding Diffusion Bonding
Tip #6: Controlling Partial Pressure Additions
Tip #7: Properly Supporting Your Work (Grids, Baskets & Fixtures)
We begin with a simple task that is often overlooked or taken for granted
Tip #1: Maintaining Your Vacuum Pumps
The heart of any vacuum furnace is its pumping system. Our goal is to keep the vacuum pumping system operating at peak efficiency. Here’s a look at how we can do this.
First, to facilitate maintenance activities with any component on a vacuum system, but most especially the pumps, it is helpful to have a running hour meter installed to document the number of operating hours on the pump between service activities.
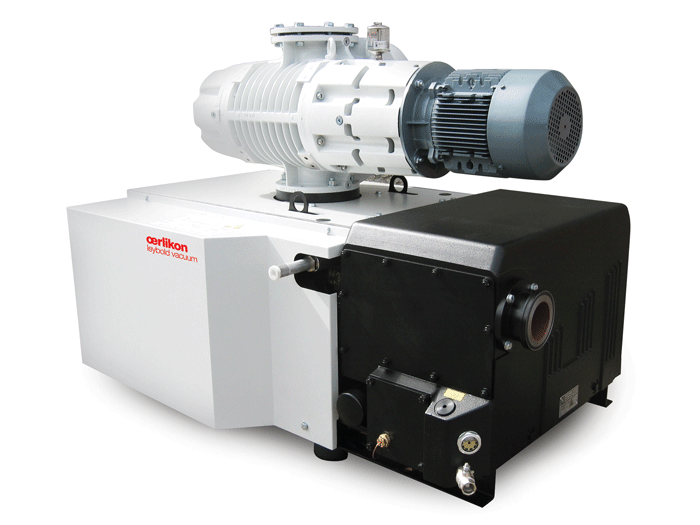
Mechanical Pumps
The mechanical (i.e. wet or oil-sealed) pump (Fig. 1) is the most common type found on most vacuum furnaces and is generally considered the workhorse of the industry. Oil-sealed pumps offer efficient, cost-effective operation and very reliable performance. However, they require a great deal of maintenance in order to continue to maintain optimum performance. This includes daily maintenance (e.g., oil level checks, ballasting), routine maintenance (e.g., oil changes) and annual maintenance (e.g., replacement of poppet valves).
Common problems [1] with mechanical pumps also require routine maintenance and inspection and include:
- Oil Contamination
- Sludge buildup
- Loose or slipping belts
- Improper oil level (too low or too high)
- Stuck discharge valve
- Clogged oil lines or valves
- Damaged discharge valve
- Ingestion of foreign contaminants (metal fins, metal chips, etc.)
- Excessive vibration (pipe connection or floor mounting)
- Exhaust filters (more than 12 months in age)
- Oil temperature not being regulated between 60ºC – 70ºC (140ºF -160ºF)
Of the various mechanical pump problems that can arise, contamination of the oil in the mechanical pump is the most common. Vapors present in the gas being pumped may condense and mix with the oil. Moisture (water vapor) is especially problematic and if not removed will flash to vapor and tie up a large portion of the pump’s gas load capacity thus creating a significant loss in pumping efficiency (resulting in either extremely long pump-down times or failure to achieve a low vacuum level or both).
In order to rid the oil of water and other liquid condensates, a gas ballast is used; a gas ballast may be used in conjunction with correctly regulating the operating temperature of the oil with a water control valve assembly; a ballast valve on the pump can be opened (manually or automatically) to admit air, nitrogen, or argon into the pump, disrupting it’s operating efficiency, the result of which is a reduction in the compression necessary to exhaust the gases and, correspondingly, a decrease in the amount of vapor that condenses. The use of a gas ballast increases the amount of oil carried out in the exhaust. The gas ballast valve is very effective in removing water vapor but actually is very ineffective in cleaning dirty oil or fixing oil that has cracked (fractionated) due to mixing with other downstream by-products.
In addition, the oil may break down chemically forming a sludge that causes numerous (short and long-term) problems with pump operation especially as it relates to severe wear on internal components, often to the point where rebuilding is not possible. Disassembly and cleaning of the pump is the only solution to this problem.
![Figure 2 [1] - Anatomy of a Mechanical Pump](https://vacaero.com/wp-content/uploads/2012/06/figure-2_lg-4.gif)
A common question asked is “How often should the oil be changed?” The most common indicators are that
- The oil looks cloudy or milky despite gas ballasting;
- The pump routinely sees a large moisture load or is used on a “wet” system.
- After a furnace bake-out (burnout) cycle has been run if you suspect that a lot of contaminants have been pulled out of the chamber and into the pump;
- If the pump will not pull down to its rated micron reading with the inlet valve shut, which is typically 0.026 – 0.067 mbar (20 – 50 microns). Note: this test is to be done using an electronic vacuum gage.
Mechanical pump oil must be changed on a routine basis (typically every 300 hours or about one month). The initial charge of oil in the pump when it is brand new should be changed after 100 hours of operation. Checking the amount of fluid in the pump reservoir during normal operation is strongly recommended. It is possible, due to improper operation, to have the pump oil backstream into the vessel in considerable quantities.
Here are some other common maintenance activities [2]:
- Check the oil level daily. There is an oil sight glass on the side of the pump where you can check the oil level. In many pumps, the oil level is designed to be at the midpoint of the sight glass (check your owners manual to be sure). If the oil level is low, add oil but be careful not to overfill the pump.
- Use the proper oil. In replacing pump oil, be careful to use the type of oil recommended for the pump and be equally careful to apply precisely the right amount of oil. Either too much or too little oil in the pump reservoir will give rise to serious difficulties.
- Change the filters. Change the filters every time you change the oil in the vacuum pump, including cleaning or replacing the filter in the oil mist eliminator.
- Lubricate bearings. The bearings in the pump should be lubricated every 3000 hours, or annual, whichever comes first.
- Inspect the vanes. The vanes (a.k.a. beater bars) in the pump should be inspected every 3000 hours, or annual, whichever comes first for cracks or worn areas. If these are found, replace as a set.
- Check the rotor rotation. Check the motion of the rotary to be sure it turns freely and make sure there is no dirt or debris in the rotor area.
- Check the motor rotation. Be sure the motor is moving in the correct direction (often this is marked on the motor by a rotational arrow). This can be done by quickly starting and stopping the motor.
![Figure 3 [3] - Dry Pump Internal Screw Assembly](https://vacaero.com/wp-content/uploads/2012/06/figure-3_lg-1.gif)
Obviously, if you hear any abnormal noises in the pump, shut it down immediately!
Dry pumps (Fig. 3) are an alternative to wet pumps especially in applications where the gas load entering the vacuum system contains vapors or particles that tend to condense or deposit in the pumping system (e.g., sintering, brazing, carburizing). These pumps can be cleaned using a high flow purge and solvent flush between periods when the pump must be partially or fully stripped down for a more thorough cleaning.
Here are the common maintenance areas on dry pumps:
- Change the filters. Change the filters every time you change the oil in the vacuum pump, including cleaning or replacing the filter in the oil mist eliminator.
- Inspect the screws. The screws in the pump should be inspected every 3000 hours, or annual, whichever comes first and cleaned thoroughly. Check for cracks or worn areas. If these are found, replace as a set.
- Lubricate bearings. The bearings in the pump should be lubricated every 3000 hours, or annual, whichever comes first.
- Check the motor rotation. Be sure the motor is moving in the correct direction (often this is marked on the motor by a rotational arrow). This can be done by quickly starting and stopping the motor.
Blowers
Vacuum blowers (a.k.a. booster pumps) typically need little day-to-day maintenance, which normally consists of simply monitoring the oil level in the pump. The blower (Fig. 4) is used in conjunction with the mechanical pump to improve pump-down rates and ultimate vacuum levels.
![Table 1 [4] - Oil Viscosity Selection Chart](https://vacaero.com/wp-content/uploads/2012/06/table_1-1.gif)
Contained within the pump housing (Fig. 5) are pistons and timing gears in a gear case fitted with troughs, which control the amount of oil, supplied to the gear teeth. A typical procedure is to fill the gear case with the appropriate grade of oil until it overflows from the oil level/filler hole. Allow the excess oil to drain before inserting the oil level filler plug. This must be done when the blower is not in operation. Overfilling must be avoided, as this will cause the gears to run hot, resulting in damage. The oil level should be checked weekly or every 100 working hours. Drain and refill the gear case to the correct level every six to twelve months depending upon the condition of the oil. When there are considerable seasonal temperature variations, it may be necessary to change the grade of oil at certain times during the year.
The oil used should be suitable (i.e. have the proper viscosity) for the minimum ambient temperature (cold starting) and for the highest oil temperature reached on maximum load (Table 1). The recommended oil for most booster pumps is straight mineral oil, which may contain anti-wear, anti-rust, anti-foam, and anti-oxidant additives. The oil should not contain either an extreme pressure additive or additives that emulsify. The oil should have a viscosity of not more than 2,500 centistokes at the minimum ambient temperature (cold starting) and not less than 30 centistokes for the highest oil temperature reached on maximum load. When there are considerable seasonal variations in ambient temperatures, summer and winter grades of oil will be required.
Typical maintenance activities for blowers include the following:
- Timing gears. Wear should be negligible over a period of years of normal service. Gear teeth are provided with the correct amount of backlash, and a reasonable amount of tooth wear can normally be accommodated without permitting contact between lobes of the two impellers. A high oil level in the gearbox will cause churning and excessive oil heating, indicated by unusually high temperature in the bottom of the sump. If the operation is continued under this condition, gears will heat and the teeth can be affected by rapid wear, which will lead to impeller lobe contact or unit seizure.
- Shaft bearings. These bearings are critical in the service life of the booster. Gradual wear may allow a shaft position to change slightly until rubbing develops between impeller and cylinder or head plate. This will cause spot heating detected by feeling these surfaces. Sudden bearing failure is usually more serious. Since the impeller shaft assembly is no longer supported and properly located, extensive general damage to casing and gears is likely to occur immediately after the bearing fails.
- Driveshaft seal assembly. This assembly consists of two individual lip-type seals submerged in oil, and located in the drive end cover, should be considered as expendable. It should be replaced as an assembly whenever oil leakage through the inboard member becomes excessive as indicated by a rise in oil level at the sight gauge. Leakage through the outboard member is not likely to be excessive unless that seal or its shaft bearing surface is damaged. Shaft smoothness and freedom from scratches have a considerable effect on the performance of this type of seal. Operating problems may also result from causes other than parts damage. Since clearances are only a few thousandths of an inch, interferences and rubs can be caused by shifts in the mounting or changes in pipe supports.
- Casing issues. Foreign materials entering the casing will also cause trouble. If this type of problem is experienced, and the casing is found to be clean internally, try removing strains. Disconnect piping and loosen mounting bolts, and reset the leveling and drive alignment. After tightening the mounting, make sure all piping meets unit connections accurately and squarely before reconnecting.
![Figure 4 [5] - Typical Roots Blower](https://vacaero.com/wp-content/uploads/2018/05/figure-4_lg-1.gif)
![Figure 5 [7] - Internal Blower Components](https://vacaero.com/wp-content/uploads/2018/05/figure-5_lg-1.gif)
Diffusion Pumps
In day to day operation, most diffusion pumps (Fig. 6) require little maintenance, only monitoring of the proper air/water cooling (e.g. flow rate, temperature, pressure), electrical power and that the pump has the proper type and quantity of fluid.
Maintaining a day-to-day log of pump and system performance (including pump-down times and ultimate vacuum level achieved) is the best indicator of the condition of the pump and provides a record of any variations that might require corrective action. In the event of a problem, confirm electrical power output, cooling water temperatures (in/out) and oil level & quality.
Common problems [1] with diffusion pumps include:
- Power failures
- Excessively high foreline pressures
- Backstreaming
- Process byproducts clogging oil returns in boiler plate
- Defective heaters and/or broken wiring on the boiler
- Water inlet temperature above 45ºC (115ºF)
- Water exit temperature above 65ºC (150ºF)
- Mixtures of hydrogen-based oils with silicone family oils
- High leak rates on the system when being pumped on
- Water-cooled copper lines full of mineral (calcium) deposits negating proper heat transfer
![Figure 6 [9] - Diffusion Pump Operation](https://vacaero.com/wp-content/uploads/2018/05/figure-6_lg-1.gif)
![Figure 7 [9] - Anatomy of a Diffusion Pump](https://vacaero.com/wp-content/uploads/2018/05/figure-7_lg-1.gif)
Of the various diffusion pump problems, exposure of the hot pump oil to the atmosphere or interruption/loss of the coolant flow is of the most concern. Accidentally introducing air when the diffusion pump is at too high a temperature almost inevitably leads to a pump malfunction or failure and oftentimes require expensive and lengthy repairs (most often at the manufacturer). Severe cracking (breakdown) of the oil and oxidation will occur, depending on the type of oil. These lead to excessive backpressure and the products of the oil breakdown will deposit on the jet structure blocking the openings or deposit in the area of the oil heater, burning it out. Overheating due to inadequate coolant flow also decomposed the oil and can cause excessive backstreaming into the vacuum furnace chamber. Depending on the actual amount of air in the hot pump, coupled with what previously deposited materials in the base of the pump, the oil may expand excessively in vapor form with a significant pressure buildup.
When servicing a diffusion pump (Fig. 7) you want to disassemble the pump so as not to damage any of the internal components, inspect the pump for damage, clean everything thoroughly and reassemble the pump properly. Common industry practice is to use acetone and then an isopropyl alcohol rinse to clean internal pump components (e.g., pump body, jet assembly). With respect to the diffusion pump fluid, remove oxidized deposits, check fluid level and color, replace fluid and have the old fluid analyzed to determine its condition (when compared to new fluid) so as to help ascertain the frequency at which it should be replaced. Reassemble the pump making sure to install new “O” rings, check for proper jet alignment, add the correct amount of new diffusion pump fluid and inspect/verify heater wiring.
Tip #2: Select The Right Vacuum Level for the Job
When selecting the right vacuum level (Fig. 7), one must ask oneself, “What type of vacuum level does my application require?” For example, while many vacuum systems using modern high-speed pumps can, in a clean, dry, empty and outgassed chamber, reach vacuum levels as low as the 10-9 torr range, we must ask ourselves, “Is this level of vacuum really necessary?” Remember, either too low or too high a vacuum level could result in undesirable surface conditions – from vaporization of elemental constituents to oxidation of surfaces causing rework or even scrap.
![Figure 1 [1] - Vacuum Level Process Comparison Chart.](https://vacaero.com/wp-content/uploads/2012/08/figure-1_lg-2.gif)
In general, applications involving vacuum heat-treating can be broken down into four main (4) categories:
- Processes that can be done in no other way than in a vacuum;
- Processes that can be done better in a vacuum from a metallurgical standpoint;
- Processes that can be done better in a vacuum from an economic viewpoint;
- Processes that can be done better in a vacuum from a surface finish perspective.
The more molecules that are removed from a vessel, the better the vacuum. The quality of a vacuum is described by the degree of reduction in gas density (i.e. gas pressure). In the field of heat-treating, processes are normally carried out in four (4) of the six (6) classifications of different vacuum levels (Table 2). Most heat treatment processes (e.g. hardening, brazing) are carried out at intermediate or so-called “soft” vacuum levels. The majority of applications are processed in this intermediate vacuum range. By contrast, at 200 miles above the earth, the vacuum in space is 1 x 10-8 torr. At 400 miles it is 1 x 10-10 torr and in deep (or outer) space is 1 x 10-16 torr.
![Table 1 [1] - Classification of Vacuum Levels](https://vacaero.com/wp-content/uploads/2012/08/table_1_lg.gif)
Notes:
[a] The SI unit of pressure is the Pascal (1 Pa = 1 N m-2)[b] Normal atmospheric pressure of 1 atmosphere is 101,325 Pa or 1013 mbar (1 bar =105 Pa)
[c] Normal atmospheric pressure of 1 atmosphere is 760 Torr (1 Torr = 133.3 Pa)
[d] Ultrahigh vacuum is defined as the pressure range between 10-6 Pa (Europe) and/or 10-7 Pa (USA) to 10-10 Pa.
Finally, an integral part of the pump selection process is detailing what you need your vacuum system to do. An important question to ask up front: “Do you need to protect the process from the pump (e.g. hydrocarbon contamination) or perhaps you need to protect the pump from the process (e.g. the ingestion of a potentially explosive or corrosive, dirt or contaminant mixture)?” Other questions for consideration may include asking yourself, “Will the pumping system only be required to pump down a chamber to a certain vacuum level and then be taken offline or will it remain at the attainable blank off pressure?” Perhaps you need the vacuum system to handle a specific mass flow while holding at a specific pressure (e.g. low-pressure vacuum carburizing). Maybe you need rapid pump down to crossover pressure of a secondary vacuum pump and then the system will be used in series to back a secondary vacuum pump such as a vapor booster or diffusion pump. Often the vacuum system will execute a pump down and then hold at vacuum under specific mass flow conditions or maybe rapid cycling from atmosphere to vacuum is required. Once these factors are determined, the right pumping system can be selected for the required vacuum level.
Tip #3: Avoid Eutectic Melting
Eutectic melting (Fig. 8) occurs when two (2) elements of a specific chemical composition melt at a lower temperature than any other composition of those elements. The temperature at which this occurs is called the eutectic temperature and the composition at that temperature produces a eutectic point (Fig. 9). For example, carbon and nickel react by melting at temperatures as low as 1326°C (2421°F) and cause localized (i.e. eutectic) melting.
Since nickel is a common element in many steels (particularly stainless steels), these materials should not be allowed to come into contact with graphite hearths or fixturing materials during heat-treating or during bake-out cycles. For this reason, graphite hearth rails are usually designed to accommodate ceramic or molybdenum inserts that separate the load from the graphite. Severe eutectic melting reactions can cause extensive damage to workloads and furnace hot zones.
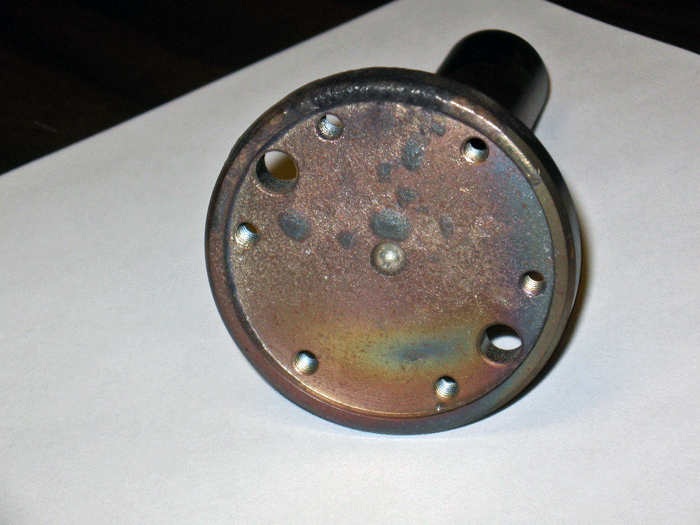
![Figure 9 [13] - Phase Diagram Depicting the Eutectic Composition, Temperature and Point](https://vacaero.com/wp-content/uploads/2018/05/figure-9.gif)
To avoid damage, it is best to understand the alloys, which you will be heat-treating and the materials of construction of your vacuum furnace. In particular, it is important to know the eutectic melting temperature (or temperature range) or any suspect combination of elements. See Reference 3 for a complete listing of eutectic melting temperatures. Once this information is known it will be possible to separate potentially reactive materials from each other by the use of completely non-reactive insulators.
There are several choices for protecting parts including high purity ceramics such as an aluminum oxide or zirconium oxide. These are often available in the form of papers, cloth blanket, plates or other solid shapes upon which the workpieces can be placed. It should be noted that some ceramic papers and cloths are hydroscopic (i.e. retain moisture) and can release fiber particles that can lead to deleterious effects on vacuum pumping systems. Ceramic materials are also available in the form of stop-off paints that can be applied liberally to furnace baskets, grids or other fixtures. These coatings must be inspected and touched up regularly as they will deteriorate with use. The same materials can be applied by plasma spray. This process provides a thicker and more durable protective finish but is slightly more expensive than the paint-on versions
Tip #4: Maintain Your O-Ring Seals
![Figure 1 [3] - Cracked "O"- Ring](https://vacaero.com/wp-content/uploads/2012/08/figure-1_lg2.gif)
O-rings are an integral part of any successful vacuum system, however, O-rings lose their elastic nature over time and eventually crack (Fig. 10) leading to air infiltration into the vacuum chamber. When replacing O-rings it is critical to use the correct type, normally specified by the original equipment manufacturer.
![Table 1 [2] - Operating Temperature Limits for O-ring Sealing Materials.](https://vacaero.com/wp-content/uploads/2012/08/table_1_lg.gif)
O-rings materials are chosen for specific locations depending on the anticipated temperature exposure during operation (Table 3). The most common general-purpose types are Buna N, Viton, and Teflon.
In order for the O-ring to function properly, it must be smooth, free of debris (Fig. 11), rounded (as opposed to flat), crack or scratch-free and properly lubricated. Whenever an O-ring seal is exposed, such as the opening and closing of the front door of a single chamber vacuum furnace, the O-ring should be wiped clean prior to each run. Carefully running one’s finger in a tight-fitting latex glove over the O-ring surface is a good way to detect minute defects or particles (e.g. dirt, metal fines) on the surface. The O-ring should be wiped clean with alcohol using a clean soft cloth then high-quality silicone vacuum grease applied in a very light coating to aid in sealing. Despite what one may think, excess vacuum grease does not aid in sealing and should be removed, as it is a getter for shop debris, which will interfere with the O-ring’s ability to seal.
![Figure 2 [3] - Debris Ladden Door Seal.](https://vacaero.com/wp-content/uploads/2012/08/figure-2_lg-2.gif)
O-ring Failures [17]
O-ring seals often fail prematurely in applications because of either improper design or material selection. From the end user’s point of view, a seal can fail in three (3) general ways:
- Leaking
- Contamination
- Change in Appearance
Contributing factors are pressure/vacuum induced stress and thermally induced stress. Elevated temperatures may cause seal degradation, swelling or outgassing. Pressure or vacuum environments (or altering between the two) can cause outgassing and weight loss.
O-ring failures can be classified into the following general categories:
- Abrasion (Fig. 12a) – The seal or parts of the seal exhibit a flat surface parallel to the direction of motion. Loose particles and scrapes are often found on the seal surface. Contributing factors include; rough sealing surfaces; excessive temperature; process environment containing abrasive particles; dynamic motion and poor elastomer surface finish.
- Flattening or Over Compression (Fig. 12b) – The seal exhibits a flat-sided cross-section, the flat sides corresponding to the mating seal surfaces. Contributing factors include: excessive compression; excessive temperature; an incompletely cured elastomer; elastomers with high compression set and excessive volume swell.
- Degradation – The seal exhibits blisters, cracks, pits, void or pockmarks on its surface. Absorption of gas at high pressure and the subsequent rapid decrease in pressure. The absorbed gas blisters and ruptures the elastomer surface as the pressure is rapidly removed. Contributing factors include: rapid pressure changes; low-modulus/hardness elastomers and incompatibility with the pressure/vacuum or thermal environment.
- Extrusion (Fig. 12c) – The seal develops ragged edges (generally on the low-pressure side) that appear tattered. Contributing factors include: excessive clearances; excessive pressure; low-modulus/hardness elastomers, excessive gland fill; irregular clearance gaps, sharp gland edges and improper sizing.
- Installation damage (Fig. 12d) – The seal or parts of the seal may exhibit small cuts, nicks or gashes. Contributing factors include: Poor techniques; improper tools, sharp edges on glands or components; improper sizing of elastomer; low-modulus/hardness elastomer and elastomer surface contamination.
- Over-compression (Fig. 12e) – The seal exhibits parallel flat surfaces (corresponding to the contact areas) and may develop circumferential splits within the flattened surfaces. Contributing factors include: improper design (failure to account for thermal volume changes or excessive compression).
- Thermal Degradation – The seal may exhibit radial cracks located on the highest temperature surfaces. In addition, certain elastomers may exhibit signs of softening—a shiny surface as a result of excessive temperatures. Contributing factors include: elastomer thermal properties; excessive temperature excursions or cycling.
- Plasma Degradation (Fig. 12f) – The seal often exhibits discoloration, as well as powdered residue on the surface and possible erosion of elastomer in the exposed areas. Contributing factors include: Chemical reactivity of the plasma. Ion bombardment (sputtering). Electron bombardment (heating). Improper gland design. Incompatible seal material.
- Other – Spiral failure (Fig. 12g) in which the seal exhibits cuts or marks which spiral around the circumference. Contributing factors include: difficult or tight installation (static); slow reciprocating speed; low-modulus/hardness elastomer; irregular O-ring surface finish (including excessive parting line); excessive gland width; irregular or rough gland surface finish and inadequate lubrication.
Figure 12 [16] – Typical O-ring Failures
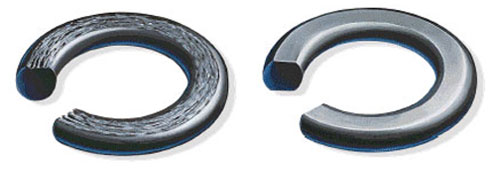
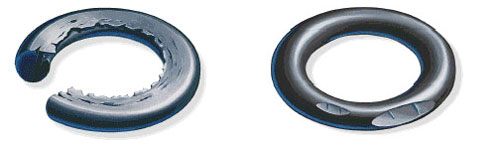
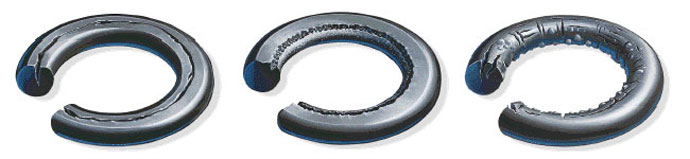
Common Misconceptions
Here are some of the most common misconceptions when working with O-rings:
- One of the most common beliefs is that the vacuum grease applied to the O-ring surface is responsible for actual sealing and that the more grease present, the better (and longer) the seal will last. In reality, the layer of vacuum grease is intended only to act as a lubricant to seat properly under the applied compression forces and should be a very thin layer, so much so that when you move your finger along the O-ring surface it will slide or glide unhindered with no appreciable amount of grease build-up.
- O-rings do not need to be re-greased after every run. False. O-rings should be wiped clean followed by running a finger (protected by a latex glove) over the surface of the O-ring to feel for damage. This is an excellent way to detect minute particles of dirt or grit and reveal nicks or areas that should be cleaned and re-greased. Thoroughly cleaning the surface of the O-ring with alcohol, methyl-ethyl-ketone (MEK) or acetone before re-greasing (or applying vacuum grease initially) is critical. Be careful to use only a company-approved solvent.
- The shelf life of a typical O-ring is forever. In reality, O-rings have a shelf life of only about six (6) months.
- O-rings cannot be spliced. Yes, they can as long as the part line is in the plane of the cross-section. They must be joined together by gluing the material with a proper adhesive.
Tip #5: Diffusion Bonding
Diffusion bonding is a solid-state joining process involving both vacancy and interstitial diffusion of atoms between surfaces in intimate contact with one another. The process is capable of intentionally bonding together a wide range of metal and/or ceramic part combinations, forming strong metallurgical bonds. Applications include hot compacting of oxides, nitrides, borides, carbides, sulfides and their mixtures to near theoretical densities as well as sintering ceramics and powder metals.
![Figure 13 [20] - Typical Hot Press Furnace](https://vacaero.com/wp-content/uploads/2018/05/figure-13.gif)
Diffusion bonding processes often involve vacuum hot pressing of multiple stacked component layers. Since many metals are easily oxidized, the process is commonly run in specialized vacuum furnaces (Fig. 13) operating in the range of 10-2 to 10-5 torr or in a special partial pressure atmosphere. A typical hot-press furnace system can operate at temperatures from 500°C – 1650°C (930°F – 3000°F) with up to 15 tons (30,000 lbs.) of compacting force. Other systems are capable of extending the maximum operating temperature, pressure and vacuum levels.
In diffusion bonding, the metal parts are held together under an applied force while they are heated, causing atoms from each part to diffuse to the other. Unlike brazing, no filler alloy is used. Diffusion Bonding is used instead of brazing for many end-use applications where the use of a filler alloy might compromise intricate features and/or vacuum integrity of components. It is also used where the temperatures of the end-use application are very high and there would be the risk of the alloy material’s softening and the joint’s weakening in service.
Pressing conditions depend on the type of metal matrix, for example, bonding composite copper – tungsten wires involves temperatures of 700°C (1300°F), pressures of 100 MPa, and times of around 600 seconds while bonding composite aluminum – boron fiber involves temperatures of 450°C (840°F), pressures of 40 MPa, and times around 600 seconds.
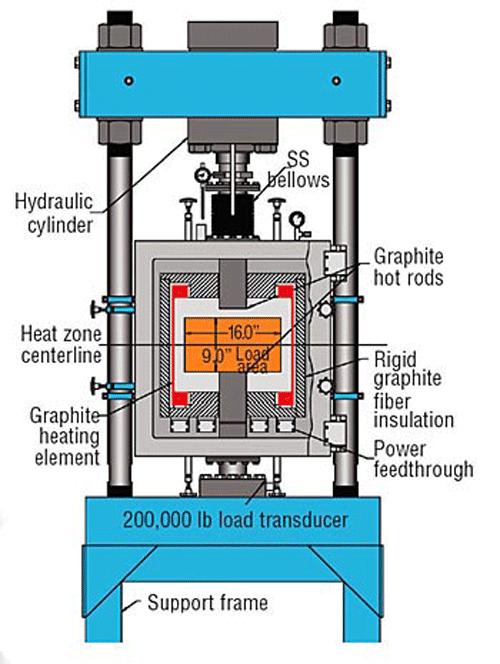
The five key parameters required for effective diffusion bonding are:
- Cleanliness of the parts
- An inert or oxygen-free atmosphere
- The unilateral application of force holding the components in contact with each other
- Temperature
- Time at temperature
In those instances, however, where our intent was not to join components together, diffusion bonding can be an unexpected problem.
In vacuum processing, metal surfaces remain very clean and free of oxides. When these near-perfect surfaces are in contact with each other or other surfaces (e.g. baskets or fixtures), certain elements have a tendency to interact between these surfaces via solid-state diffusion (i.e. interstitial diffusion of atoms across the interface). The result is that the parts “stick” together or stick to the baskets or fixtures, the equivalent of being welded on a microscopic level. In some cases the effect is minor and a slight tapping of the components separates them and the surface “damage” is inconsequential. In other cases, parts are fused together so strongly that the surfaces have to be literally ripped apart, ruining the components.
In most metals, the presence of oxide layers at the surface will affect the ease of diffusion bonding. For some metals and alloys, their oxide films either dissolve in the bulk of the metal or decompose at the process temperature (e.g. steel, copper, titanium, tantalum, columbium, and zirconium), and so metal-to-metal contact can be readily established at the interface (Fig. 15). However, if the oxide film is chemically stable, as for aluminum alloys, then the chances for diffusion bonding to take place are limited.
The loads involved are usually below those that would cause macro-deformation of the parent material(s). Temperatures of 50% to 90% of the absolute melting point (0.5-0.9Tm where Tm is the melting point in ºK) are needed. Time at temperature for the effect to take place can range from as little as one (1) minute to sixty (60) minutes or more. This depends upon the materials being bonded, the joint properties required and the remaining bonding parameters.
An example of diffusion bonding is when loads of saw blades or knives are packed closely together in fixtures so as to minimize distortion during vacuum processing. This pressure, in combination with high temperature, is enough to cause sticking to occur, even though partial pressures of nitrogen are commonly used. In most instances, the parts can be tapped gently and separated. In extreme cases, they bond together and must be scrapped. To negate the effects of diffusion bonding, partial pressure levels can be set high enough to prevent inter-surface diffusion or coatings (e.g. magnesium oxide or boron nitride) can be applied to the part surfaces in question to prevent sticking from occurring.
![Figure 15 [23] - The Mechanism of Diffusion Bonding](https://vacaero.com/wp-content/uploads/2018/05/Figure-3a.gif)
![Figure 15 [23] - The Mechanism of Diffusion Bonding - (a) Initial 'point' contact, showing residual oxide contaminant layer](https://vacaero.com/wp-content/uploads/2018/05/Figure-3b.gif)
![Figure 15 [23] - The Mechanism of Diffusion Bonding](https://vacaero.com/wp-content/uploads/2018/05/Figure-3c.gif)
![Figure 15 [23] - The Mechanism of Diffusion Bonding -](https://vacaero.com/wp-content/uploads/2018/05/Figure-3d.gif)
Tip #6: Controlling Partial Pressure Additions.
Many of today’s vacuum furnaces are equipped with a partial pressure control system, which can be designed to operate with a single gas or multiple gases of various types – for example, nitrogen, argon, hydrogen, and helium. Partial pressure is typically programmed into the furnace temperature controller (Table 4), activated as one of the programmed events.
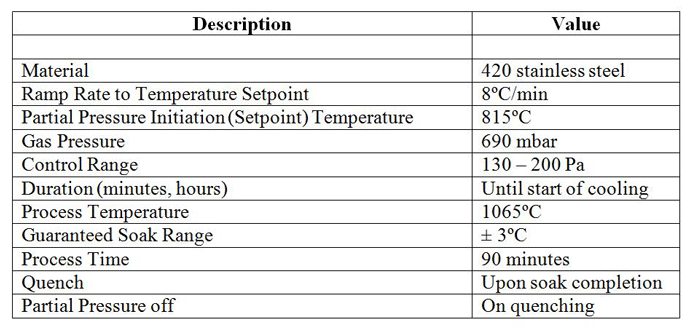
Why Use Partial Pressure?
Introducing a partial pressure gas into a vacuum furnace at a pressure in excess of the materials vapor pressure will help avoid significant evaporation or “boiling away” of elemental constituents in the materials being processed. Without this control, surface integrity and in some cases the chemical composition of metal (or filler metal) can be affected.
The primary purpose of a partial pressure system (Fig. 16) is to avoid vaporization (i.e., boiling away) of elemental constituents, chromium as an example, from the component parts being run. Partial pressure also minimizes diffusion bonding (i.e. sticking or fusing of part surfaces together) that can take place on adjacent metal surfaces. In the case of brazing, it also serves to minimize or prevent vaporization of the constituents in the braze alloy. A secondary purpose of partial pressure control is to dilute and help sweep away unwanted vapors that may be present when outgassing occurs during heating. In most cases, however, a properly designed and robust pumping system will remove any outgassed vapors before the partial pressure setpoint is reached.
Stainless steels, tool steels, and more exotic alloys are often processed in vacuum. If chromium is present in these materials it can evaporate at temperatures and pressures within normal heat treatment ranges. When processing above 990°C (1815°F) chromium will vaporize if the vacuum level is less 1 x 10-4 torr and/or parts are held for a prolonged time. Heat treaters often observe a greenish discoloration (chromium oxide, Cr2O3) on the interior of their vacuum furnaces, the result of chromium vapor reacting with air leaking into the hot zone. Otherwise, the evaporation deposit is bright and mirror-like. To avoid this, an operating partial pressure between 0.3 and 5 torr is typical for most chromium bearing steels.
For vacuum brazing (silver, copper, nickel) depletion of the filler metal alloy can be avoided by raising the pressure in the furnace to a level above the vapor pressure of the alloy at brazing temperature. For example, copper having an equilibrium vapor pressure at 1120°C (2050°F) of 1 x 10-3 torr is usually run at a partial pressure between 1 and 10 torr. Nickel brazing normally is done in the 10-3 to 10-4 range. However, in the 10-5 to 10-6 torr regime, you run the risk of losing some of the nickel which has an equilibrium vapor pressure of 1 x 10-4 torr at 1190°C.
How Do Partial Pressure Systems Work?
The partial pressure system functions in the following manner. The partial pressure function is programmed into the cycle recipe. During the cycle, a pressure valve opens when the preprogrammed event setpoint is reached. Gas is introduced into the chamber and controlled within a pre-established range. Typical partial pressure settings for vacuum heat treatment are in the range of 65 – 200 Pa (500 – 1500 microns) depending on the material and process being run, but the setpoint value can, in general, be as low as 13 Pa (100 microns) and as high as 665 Pa (5000 microns) if desired (and if appropriate cautions are observed). A bypass circuit on the pumping system is used so that the gas pressure can be held in the desired setpoint value. It should be noted that the diffusion pump is not utilized, and the high vacuum valve is closed.
In some systems, a micrometer needle valve is also provided, often located in the line adjacent to the partial pressure solenoid valve, so that the pressure in the vacuum chamber can be maintained within closer setpoint limits than a system without such a device.
Another use of “partial” pressure is during convective heating, which takes place anywhere from sub-atmospheric pressure (in the range of 6.5 Pa (50 microns) or higher) all the way to a positive pressure in the range of 1 – 2 bar. Typically for a circulation fan to effectively move gas molecules, the pressure range must be a minimum of 65Pa (500 microns) or higher. After pumpdown, the furnace is backfilled to the selected pressure and heating begins. In this instance, partial pressure is used to reduce heat-up time with heavy loads and improve temperature uniformity throughout the load.
Hydrogen Partial Pressure
Special consideration and NFPA specification requirements should be followed for partial pressure systems involving combustible gases, an example of which is a hydrogen partial pressure system. One of the important prerequisites to running hydrogen is to make sure that the vacuum furnace is “tight”, that is, that the unit has a leak rate in the range of 2 – 5 microns per hour (maximum). This can be done manually, or in some systems, it is performed automatically when hydrogen partial pressure is selected as part of the program recipe. As such, a leak check (see below) should be performed before every cycle involving the introduction of hydrogen. In addition, while hydrogen or any other combustible gas (e.g. acetylene, propane, etc.) is in use, the furnace should not be left unattended.
One of the other safety features required on the equipment is that the mechanical pump must be equipped with a (nitrogen or argon) purge system to dilute the hydrogen exiting the vacuum vessel out the pump exhaust so that it is non-combustible. In the case of a hydrogen partial pressure circuit, it is common to find a micrometer needle valve on the partial pressure line to minimize the oscillation around the desired setpoint. In addition, the upper setpoint limit is often reduced to a maximum of 1500 microns.
Today, inert gas safety purge systems are another feature commonly provided and activated (automatically or manually) in specific situations to dilute the combustible gas mixture. Another feature is the use of dual pressure relief valves on the vacuum furnace chamber (piped to exhaust outside the building) a common option offered by equipment manufacturers. Combustible gases are considered safe to use so long as the delivery systems, distribution systems, removal systems, and equipment are robust and “explosion-proof”. National Fire Protection Agency (NFPA) standards should be consulted and the vacuum furnace designed in accordance with NFPA 86.
Which gases can we use?
The use of partial pressure of hydrogen in vacuum furnaces, as both a process gas (for example in re-brazing applications) and especially for furnace and fixture cleanup cycles, is increasing in popularity once again. Safety interlocks on the furnace and control of the delivery, use, and discharge of hydrogen throughout the operation are MANDATORY to ensure both employee and equipment safety.
Argon and nitrogen are the most common partial pressure gases. Often, argon is selected since it tends to “sweep” the hot zone, that is, being a heavier molecule it tends to reduce evaporation more effectively than say nitrogen (or hydrogen). Specialized applications such as those in the electronics industry may use helium or even neon (if an ionizing gas is needed). Gases with a minimum purity of 99.99% and a dewpoint of -60°C or lower should be specified.
Certain other cautions are also in order. For example, nitrogen may react with certain stainless steels or titanium bearing alloys resulting in surface nitriding. In the case of hydrogen, the normally near-neutral vacuum atmosphere can be shifted sharply to a reducing atmosphere and embrittlement by hydrogen is a concern for certain materials such as titanium, tantalum, and zirconium.
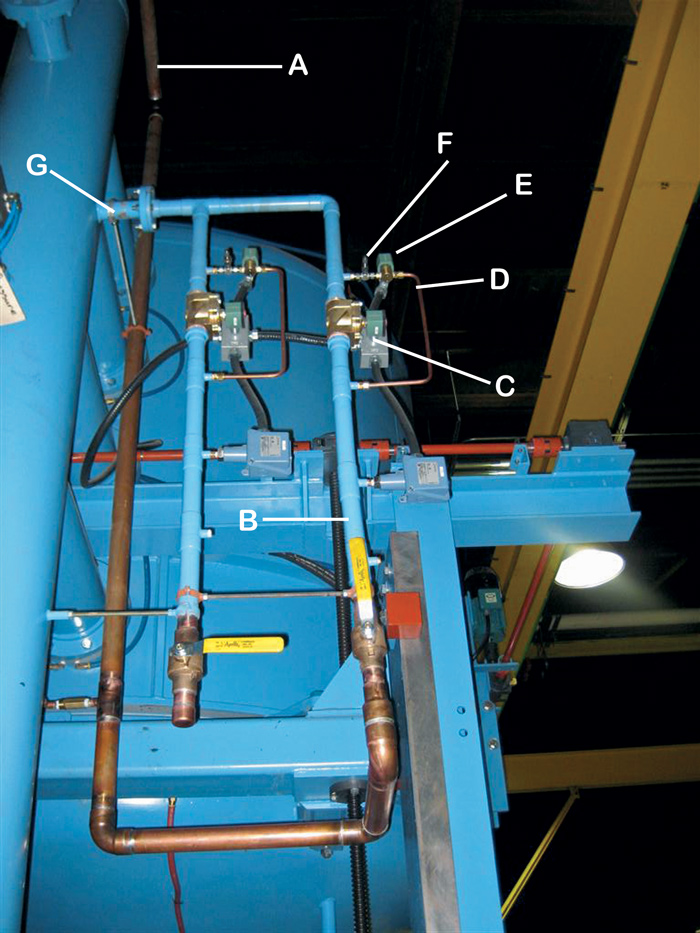
Key:
A: Incoming Gas Supply Line
B: Backfill Line No. 1
C: Quench Solenoid
D: Partial Pressure Line
E: Partial Pressure Solenoid Valve
F: Partial Pressure (Micrometer) Needle Valve
G: Inlet Into Furnace
Measurement and Control
It is critical to know the exact pressure, flow, and type of gas being injected into the vacuum furnace so that the process being run is under control (Fig. 16). It is not uncommon to believe, for example, that you are running an argon partial pressure at 1 torr when in reality you are running at 0.4 torr or with hydrogen (or helium) that you are at 10 torr when you are really at 1 torr. Absolute pressure gauges should be used to determine precise partial pressure values since thermocouple gauges are affected by gas species (since they are calibrated for air).
For flow accuracy, flowmeters should have a micrometer needle valve installed in the downstream line. On many units, the gas is pulsed in using a solenoid valve and setpoint control on the vacuum gauge, akin to continuous flow with a needle valve installed. Also, it is extremely important to inject the partial pressure gas directly into the hot zone so that the gas does not short circuit the work area.
Safety Considerations for Partial Pressure Systems
Leak Checks
In simplest terms, the leak rate (aka leak-up rate) of a vacuum furnace, typically measured in microns per hour, is the rate of pressure rise when the vacuum chamber is isolated from its pumping system and held for a given length of time. Two factors contribute to the value obtained, the actual rate of leakage due to the pressure rise from leaks in the chamber and leakage due to other factors (virtual leaks, permeation, diffusion, outgassing, backstreaming, etc.). The later values typically decrease over time.
Leak checks should be done for a one-hour duration, but it is common industry practice to reduce the amount of time and multiply by the appropriate value (for example, using a 30-minute test duration one would multiply by 2 to achieve a value “equivalent” to one hour). While an hour is always recommended, under no circumstances should the time for leak checking be under 10 minutes, and if unacceptable results are obtained, the re-test time duration should be increased.
Ideally, the vacuum furnace should be “clean, cold, empty and outgassed” in order to obtain accurate values. In the real world, however, this seldom happens. What is common, however, is to run a “clean up” cycle prior to performing a leak check. However, if leak checking is done before every cycle (automatically or manually), clean-up cycles are often relegated to once a week. If the furnace fails the leak rate test, it can be repeated. If the furnace repeatedly fails, the cause of the leak should be identified, isolated and corrected.
The leak check procedure involves evacuating the chamber with the furnace at ambient temperature to the lowest possible vacuum level (i.e., the ultimate vacuum achievable), isolating the chamber by closing the main vacuum valve to the chamber and noting the beginning vacuum level a minute or two after the valve closes. Measure the vacuum level again after the chosen time interval is over and calculate the rise in pressure per unit time. Acceptable leak rates for all-metal lined vacuum furnaces are in the 1 – 2 micron/hour range, while graphite hot zones can be in the 5 – 10 micron/hour range.
Gas Hazards
When dealing with any gas which may cause asphyxia, remember that oxygen may be displaced to a level of 16% of the gas/air mixture before symptoms appear. Marked symptoms appear when the oxygen concentration has been reduced to below 16%. Death will occur in minutes when the oxygen content is below 10%. First symptoms are hunger and rapid respiration. This is followed almost immediately by diminished mental alertness and impaired muscular coordination. Later, judgment is impaired and all sensations are depressed. Emotional instability and the rapid onset of fatigue are often reported. As asphyxiation progresses, there may be nausea, vomiting, prostration, loss of consciousness, convulsions, deep coma, and death.
Gases and liquefied compressed gases may be classified as:
- Corrosive: Products that react chemically and deteriorate materials (such as skin) they come in contact with.
- Cryogenic: Contact with these liquids cause “cryo burns” due to the freezing of skin as well as vaporizing rapidly, which if in confined spaces can cause asphyxiation.
- Flammable: When mixed with air, oxygen, or another oxidant burns or explodes upon ignition.
- High Pressure: A sudden release of pressure may cause serious damage to personnel or equipment.
- Inert: These displace oxygen in confined spaces and threaten life support.
- Oxidant: Gases that initiate and support combustion.
- Toxic: Substances that may chemically produce injurious or lethal effects.
Some basic properties of gases used in vacuum systems are:
- Argon (chemical symbol: Ar). Heavier than air. Difficult to purge from pits and recessed areas.
- Nitrogen (chemical symbol: N2). Slightly lighter than air.
- Helium (chemical symbol: He). Much lighter than air.
- Hydrogen (chemical symbol: H2). Much lighter than air. Accumulates in ceiling areas and can reach dangerous concentration levels. Roof sensors tied to automatic ventilation systems are recommended.
- Methane (chemical symbol: CH4). Very slightly heavier than air. Often confused with natural gas (typically 90 – 95% methane). Natural gas has an odorant added, methane does not.
Examples of gases whose primary hazard is an explosion if an air/gas mixture should occur include:
- Ammonia. This gas has a lower explosive limit of 27% and is highly flammable.
- Hydrogen. An odorless gas that normally burns with a colorless nearly invisible flame making it very difficult to detect. The lower explosive limit (LOL) of hydrogen is 4%.
- Propane (chemical symbol: C3H8). A gas with a slight odor that is heavier than air and which can accumulate in pockets below ground level, such as pits.
Symptoms of gas poisoning
One should also be aware of the changes (either rapid or slow) in one’s physical condition. Symptoms may include:
- Dizziness
- Headache
- Stiff neck
- Weakness
- Nausea
In many instances, if a person collapses, the safety policy may tell you not to attempt to rescue the person in question until after ascertaining that the area is completely safe. Most people find this to be a very “disturbing” policy, but if you think about it for a moment you will realize that the problem that overcame a fellow worker may overcome you!
Be sure you are very clear on your company policy regarding this issue – ask questions and bring up numerous “what if” scenarios in safety meetings and remember to think before acting.
When possible, remove all personnel from, and seal off the area in question until proper ventilation is provided. In the event a person is overcome call for medical attention immediately and implement plant safety procedures.
In Conclusion.
The use of partial pressure in vacuum furnace systems can be extremely beneficial to the component parts being processed and is an option that should be provided on every new furnace and retrofit on those which do not have it now. Partial pressure is recommended for many heat treating and brazing processes to achieve the results we expect. Introduction of the partial pressure gas into the furnace hot zone at one or more locations, having the gas enter as a continuous flow stream and holding pressure over a predetermined range (rather than trying to operate at a single pressure) are important. The choice of partial pressure gas is also important both from a cost and quality standpoint.
References
1. NFPA standards (www.nfpa.com)
2. Jones, William R., Partial Pressure Vacuum Processing – Parts I and II, Industrial Heating, September/October 1997.
Tip #7: Materials Selection for Grids, Baskets & Fixtures
General Design Considerations
To obtain the most cost-effective design requires a thorough understanding of the service conditions under which the material will be exposed. Important considerations include:
- Normal operating (exposure) temperature as well as the maximum (and minimum) usage temperatures;
- Metallurgical stability over the expected duty/thermal cycle (period, frequency, and rate of heating/cooling);
- Thermal expansion characteristics;
- Fabrication (or casting) methods (with respect to the development of thermal or chemical gradients in the material);
- Design with respect to applied load(s), repetitive force and transfer of the load to the load bearing members;
- The manner of loading, type of support, and external constraints;
- Required versus desired life;
- Environment(s) to which the material will be exposed;
- Availability;
- Cost versus life.
In addition, designers must also be concerned with (a) room temperature properties (e.g. strength and ductility, machinability and weldability), (b) elevated temperature properties (e.g. hot strength, ductility and creep resistance, thermal shock and thermal fatigue resistance, hot hardness and resistance to carbon pickup), (c) physical properties (e.g. modulus of elasticity, electrical resistivity, coefficient of thermal expansion and thermal conductivity); (d) operating environment (e.g. type of quenchant, exposure of partial pressure species such as carbon or nitrogen, sulfur or chlorine, etc.) and perhaps (e) cast properties (e.g. fluidity).
Alloy Materials
High-temperature alloys encompass both cast and wrought products that are available from a number of qualified suppliers. Heat resistant alloys are primarily selected for their ability to perform a function over a wide range of application temperatures. However, the measure of performance is different in each case and varies as the use factors change.
In many applications, high temperature creep data (e.g. 1% creep in 10,000 hours) and/or stress rupture data provided by manufacturers is the best comparison of the differences between alloys used for heat treating applications. Over time, however, creep may lead to excessive deformation, and even failure (fracture) at stress levels much lower than those determined at room temperature and at elevated temperature short duration tensile conditions. When the degree (rate) of deformation is the limiting factor, the design stress at temperature should be chosen below that which produces that limiting rate (limiting creep stress) or below the stress which will produce that limiting degree of deformation in a given time.
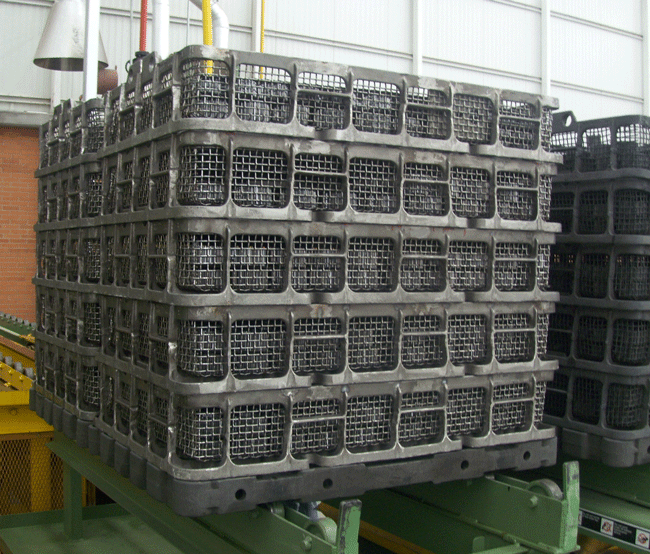
Carrier or base grids are available in a number of styles including serpentine, cast alloys and fabricated wrought alloy designs. Choice of design is a function of application and is primarily dependent on hot strength, that is, the measure of strength at the operating temperature and the ability to resist deformation, or attack of various gases present in the vacuum system in a given thermal environment and creep, a measure of a combination of failures such as distortion, thermal fatigue, thermal shock, carburization, and elemental attack on the metal matrix.
Selection, loading, and maintenance of baskets and fixtures (Fig. 17) are equally important. Selection of the basket material is often (but not always) influenced first by cost, then service environment and finally compatibility with the workpiece and furnace hearth.
Common in the industry are rod framework baskets constructed from alloy bars typically 9.5mm – 16 mm (0.375” – 0.625”) in diameter, with woven wire mesh liners. For vacuum service temperatures to 980°C (1800°), austenitic stainless steels (e.g. 304, 309, 310 and 330) are commonly used. However, these alloys can become embrittled from long exposure to temperatures in the range of 595°C to 815°C (1100°F to 1500°F) due to carbide precipitation and sigma formation. In this temperature range, more expensive alloys such as 35Ni-15Cr or Inconel® 600 have been reported to be more stable and may justify the extra cost. For even higher temperatures, alloys like Haynes 230 or MA 956 (a nickel-based oxide-dispersed alloy) or molybdenum alloys are reported to provide good life. Care must be taken in handling any baskets but especially those made from molybdenum since they become brittle after initial exposure to high temperatures.
A fine grain size is preferred to resist thermal fatigue damage (ASTM 5 or finer). If welded, fully penetrated joints are necessary such as by pressure welding (cross-wire resistance welding) the frame members.
Graphite Materials
For certain vacuum applications, graphite fixtures (Fig. 18) are preferred due in large part to the strength of graphite, which increases with temperature and graphite’s good thermal shock resistance, conductivity and the fact that it is relatively inexpensive.
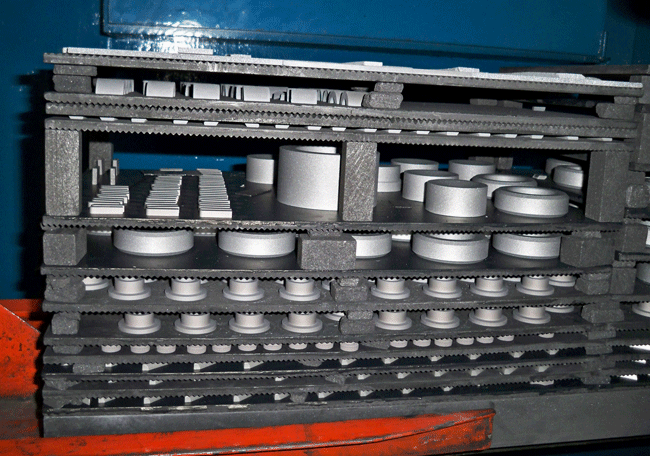
Graphite fixtures are typically used by the heat treating industry in the form of solid flat plates, plates with milled cavities or plates with holes for passage of cooling gases. Normally the thickness for solid plates will range from 3mm – 19 mm (0.125” – 0.75”) while milled plates can be up to 100 mm (4”) or thicker. Many graphite fixtures are designed to be stackable.
Graphite fixtures should be made of high-purity, high-density graphite. It is difficult to cite specific property levels for commercially available carbon and graphite materials since each manufacturer offers a variety of sizes, shapes, and grades depending on the application, thermal conditions, fixture design, loading, life expectancy, and the amount of labor required for loading. When the price is an issue, and the application does not require extended life, medium grades or isostatically pressed lower grades of graphite may be considered. Ultra-fine (ultra-high purity) and super dense grades of graphite can significantly extend the life of a graphite fixture.
Carbon/Composite Materials
Carbon/carbon composite (C/C) fixtures and grids (Fig. 19) have become an attractive alternative to conventional alloy materials. A carbon/carbon composite contains from two (2) primary components, carbon fibers and a carbon matrix (or binder). Carbon fibers are extremely thin, typically 0.005 mm – 0.010 mm (0.0002” – 0.0004”) diameter and are strands of carbon atoms. They are interlaced in such a way as to provide mechanical strength, stiffness, and thermal conductivity. The carbon matrix allows for uniform weight transfer and chemical resistance to attack. Properties can vary depending on whether they are measured parallel or perpendicular to the surface.
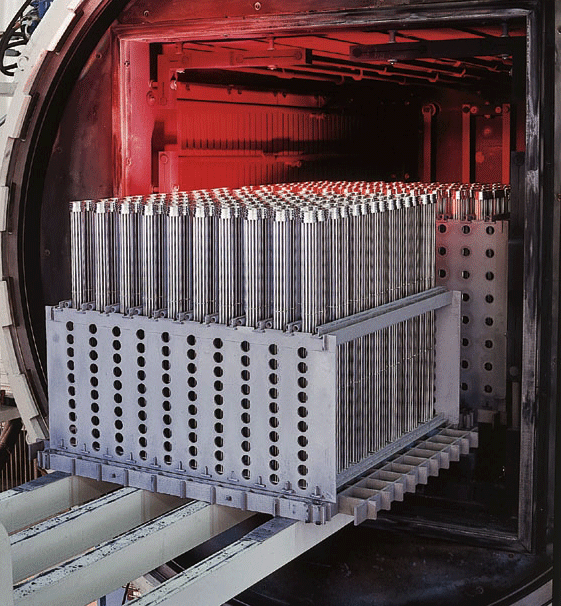
Carbon/carbon composite material has low thermal mass (specific heat), high strength-to-weight ratio at temperature and negligible thermal deformation creating favorable net/tare weight ratios. This allows for rapid heating and cooling rates, heavy part loading, and, in many cases, improved part distortion. C/C also has excellent fatigue resistance minimizing issues with crack propagation.
As with graphite materials, each manufacturer offers a variety of sizes, shapes, and grades. Purity levels in the range of 300 ppm total impurities are acceptable for general purpose heat treating with specialty applications available to as low as 10 ppm. A fully densified C/C material will be around 1.50 g/cm3 or higher. Once again, it is important to view all properties together when evaluating the quality of a composite material.
It should be noted that eutectic melting is possible at temperatures exceeding 1050°C (1922°F) but this is highly dependent on the alloy being treated. For example, it has been reported that while melting of certain tool steels (e.g. M50) has occurred when placed in direct contact with C/C materials other materials such as superalloy turbine blades processed at temperatures in the range of 1290°C (2350°F) show no reaction. Ceramic barrier layers or alloy mesh screens can be used to avoid this problem. Care must also be taken when attempting to unload these materials into open air at temperatures above 350°C (662°F) as C/C readily oxidizes over time thus destroying or severely degrading mechanical properties.
Low-pressure vacuum carburizing with high-pressure gas quenching is an example of where C/C fixtures and/or combination designs with alloy posts are common. The oxygen-free vacuum environment coupled with inert quenching gases avoids surface reactions with the fixtures.
In Conclusion.
As a whole, the heat treat industry must become better educated on materials for grids, baskets, and fixtures in order to extend the useful life of these components. We must be able to fully understand how to apply the alloys we have and work as partners in the development of new alloys to keep pace with the changing nature of the heat-treating industry. In particular, more sharing of practical information about high-temperature material performance over the full spectrum of heat-treating applications is needed throughout the industry.
For his part, the heat treater must keep better records of the service history of his grids, baskets, fixtures, and internal furnace components including a history of duty cycles as a function of the application, performance life and failure modes of the alloys. For their part, the alloy fabricators and casters must help interpret this field data, adding their technical expertise on material design and help design more meaningful tests.
References
- Herring, D. H., Vacuum Heat Treatment, BNP Custom Media Group, 2012.
- Control Specialties (www.control-specialties.com)
- Zoellig, Uwe and Mario Vitale, Dry-Compressing Vacuum Pumps Increase Productivity and Reduce Operating Costs in Demanding Heat-Treatment Applications, Industrial Heating, January 2010.
- Blower Maintenance Manual DRI-114-0502, Dresser Roots
- Dresser Roots (www.dresserroots.com)
- RGS HVB High Vacuum Booster Installation, Operation & Maintenance Brochure ILRB-3008 rev 0510, Dresser Roots.
- Know How, Vacuum Generation, Pfeiffer Vacuum (www.pfeiffer-vacuum.com).
- McCarthy, David, Diffusion Pumps for Vacuum Furnace Applications, Agilent Technologies Inc., 2009.
- Vacuum Furnace Operation and Maintenance, Ipsen-U, 2009.
- Herring, Daniel H., Vacuum Heat Treatment, BNP Custom Media Group, 2012.
- Luby, Jeffrey P., How to Select the Right Pump for the Application, Industrial Heating, 2006.
- Critical Melting Points and Reference Data for Vacuum Heat Treating, Osterman, Virginia and Harry Antes, Jr. (Eds.), Solar Atmospheres, Inc., September 2010.
- The Vacuum Technology Book, Volume 1, Pfeiffer Vacuum
- Herring, Daniel H., Vacuum Heat Treatment, BNP Custom Media Group, 2012.
- Moyer, Michael, Keeping it Bright, ASM International Vacuum Maintenance Seminar, Anaheim, CA, 2008.
- Herring, Daniel H., The Ubiquitous O-Ring, Industrial Heating, November 2009.
- Marco Rubber & Plastic Products, Inc. (www.marcorubber.com), private correspondence.
- The Vacuum Technology Book, Volume 1, Pfeiffer Vacuum (www.pfeiffer-vacuum.net)
- Solar Atmospheres (www.solaratm.com)
- Centorr Vacuum Industries (www.vacuum-furnaces.com)
- Rogers, Bill, Hot-Press Furnace Systems Handle Extreme Operating Conditions, Industrial Heating, September 2004.
- McCurdy, Kevin J., Vacuum Furnace Maintenance – Brazing and Sintering, ASM International Vacuum Maintenance Seminar, Anaheim, CA, 2008.
- Vacuum Process Engineering (www.vpei.com)
- Herring, Daniel H., Vacuum Heat Treatment, BNP Media II, 2012.
- Jones, William R., Partial Pressure Vacuum Processing – Part I and II, Industrial Heating Magazine, September/October 1997.
- Fabian, Roger, ed., Vacuum Technology: Practical Heat Treating and Brazing, ASM International, 1993.
- Practical Vacuum Systems Design, The Boeing Company.
- Herring, Daniel H., Vacuum Heat Treatment, BNP Custom Media II, 2012.