D. Scott MacKenzie, Houghton International Inc. Valley Forge, PA
The heat treatment of landing gear is a complex operation requiring precise control of time, temperature, and carbon control. Understanding the interaction of quenching, racking, and distortion contributes to reduced distortion and residual stress.
Arguably, landing gear has perhaps the most stringent requirements for performance. They must perform under severe loading conditions and in many different environments. They have complex shapes and thick sections. Alloys used in these applications must have high strengths between 260 to 300 ksi (1,792 to 2,068 MPa) and excellent fracture toughness (up to100 ksi in.1/2, or 110 MPa×m0.5). To achieve these design and performance goals, heat treatments have been developed to extract the optimum performance for these alloys.
Alloys Used
The alloys used for landing gear have remained relatively constant over the past several decades. Alloys like 300M and HP9-4-30, as well as the newer alloys AF-1410 and AerMet 100, are in use today on commercial and military aircraft. Newer alloys like Ferrium S53, a high-strength stainless steel alloy, have been proposed for landing gear applications. The typical chemical compositions of these alloys are listed in Table 1.
The alloy 300M (Timken Co., Canton, OH) is a low-alloy, vacuum-melted steel of very high strength. Essentially it is a modified AISI 4340 steel with silicon, vanadium, and slightly greater carbon and molybdenum content than 4340. The alloy is governed by standard AMS 6417. This alloy has a very good combination of strength (280 to 305 ksi, or 1,930 to 2,100 MPa), toughness, fatigue strength, and good ductility. It is a through hardening alloy to large thicknesses.
HP9-4-30 (Timken Co.) is a low alloy, high strength steel with a high nickel and cobalt content for toughness. The typical tensile strength is approximately 240 ksi (1,655 MPa). It is produced by consumable electrode vacuum arc remelting process. Production is governed by standard AMS 6526.
AF1410 (Timken Co.) is a very high-strength steel that exhibits excellent fracture toughness properties. It is typically used in the 260 ksi (1,790 MPa) tensile strength range. It is double vacuum melted (vacuum induction melted followed by vacuum arc remelting), for extremely low inclusions and homogeneity.
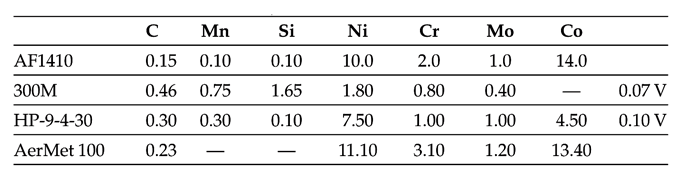
Ferrium S53 (Questek Innovations LLC, Evanston IL) is a martensitic, carbidestrengthened, secondary-hardened corrosion resistant steel developed to serve as a drop-in replacement for 300M steel. Stress corrosion cracking testing has shown performance equivalent to PH15-5, but with strengths up to 290 ksi (1,999 MPa).AerMet 100 (Carpenter Technology Corp., Reading, PA) is a high strength, high toughness alloy, with excellent resistance to stress corrosion cracking and fatigue. It is usually used in the 875 and 925ºF (470 and 495ºC) aging treatment condition.
Billets of the desired alloy are forged in complex closed dies to a general overall shape, and the forgings are allowed to air cool at the forge shop. Generally, these forgings are then shipped to the manufacturer for machining. The forgings can be very large. For example, the raw forging for an U.S. Navy F-18 C/D landing gear weighs about 830 lb (380 kg). To give some idea of how much machining may be required; this forging weighed about 110 lb (50 kg) after machining. In a typical commercial aircraft, the main landing gear is approximately 10 ft tall, with the maximum cross section of between 4 and 5 in. (3 m and 100 to 127 mm), and part weight can be upwards of 1,500 lb (680 kg).
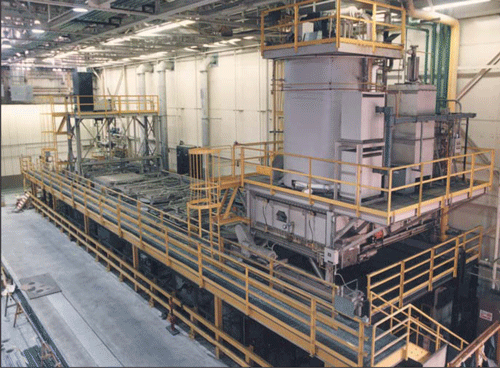
Fabrication Sequence
The forging is net shape after machining. No other machining other than honing the central bore is performed. The components are then heat treated, and the part is inspected using magnetic particle inspection. A dimensional check of the distortion from heat treatment is also monitored. The bore is honed, and the part is thoroughly cleaned for surface plating or coating. Multiple stress-relieving thermal treatments are applied throughout the process, and especially after plating. The part is then inspected again, and the final protective coat of paint is applied. Once a landing gear set has been completed, it is moved to final assembly for assembly into a ship-set.
Heat Treatment of Landing-Gear Alloys
Historically, the heat treatment of landing gear has been done in large gantry-type atmosphere furnaces (Fig. 1). Endothermic atmosphere produced by a gas generator, or by in-situ production of endothermic gas by dissociation of methanol have been used, and both methods are still in use today. The dissociation of methanol using an exterior generator also has been used. Because of the design of the furnaces, it is difficult to place an internal fan inside the furnace, and stratification of the atmosphere can become a problem.
The successful control of the atmosphere produced for the neutral hardening process depends on the accurate prediction and regulation of the carbon potential of the atmosphere inside the furnace. This balances the effective carbon in the steel with the carbon potential of the atmosphere so that no decarburization or carburization occurs on the parts. In production furnaces, the exact composition is difficult to predict or calculate because of air infiltration, oily work, and residual coolant on work pieces, etc. By experience, it is possible to achieve good carbon control. A properly calibrated carbon or oxygen probe will provide an accurate representation of the carbon potential if the atmosphere is stable or near equilibrium.Control of the atmosphere is critical. Because the heat treatment of landing gear is a neutral process (neither carburizing nor decarburizing), precision carbon control is essential. Because of the nature of the alloys, and the high strength levels required, regions of decarburization serve as a weak spot and can be the cause of the initiation of low-cycle fatigue. Carburization also can be an initiation site for fatigue or stress-corrosion cracking. In general, decarburization is limited to a partial decarburization of 0.003 in. (0.076 mm) maximum. Complete decarburization is prohibited. Carburization is limited to 20 KHN increase over the core hardness. Because of the difficulty and expense of testing individual landing gear for decarburization or carburization, multiple test specimens accompany the parts throughout the process. Test slugs for carbon measurement, and test blocks for fracture toughness and tensile specimens typically accompany each part. The test blocks are serialized so they can be identified with a specific landing gear component, so that if a problem occurs, the landing gear can be identified and quarantined for disposition.
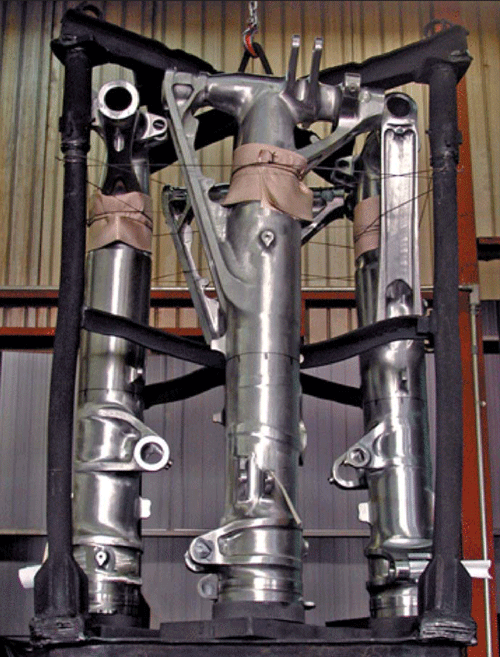
For the atmosphere to be neutral to the steel, the activities of the carbon in the atmosphere and the steel must be equal. It is the activity of the atmosphere that is directly indicated and controlled by the carbon probe. The carbon activity in steel depends on the temperature and the concentration of carbon, as well as the concentration of alloying elements. Because aerospace alloys contain significant concentrations of alloying elements, it is important to consider the various effects of the various elements if successful neutral hardening is to be accomplished. In general, silicon, manganese, cobalt, and nickel tend to cause the activity of carbon in steel to increase, while the elements chromium, molybdenum, and vanadium tend to decrease the activity of carbon in steel. For 300M, the carbon potential to achieve neutral hardening tends to be approximately 0.08% higher at the typical austenitizing temperature of 1600ºF (870ºC). This would mean that the carbon potential in the furnace would have to be approximately higher by 0.08% than the carbon content in the steel would indicate.
Routine weekly testing with alloy test slugs are used to validate the atmosphere, along with routine daily-by-shift shim stock analysis. Shim stock testing and the test slug are typically logged in a statistical process control chart, with variations immediately corrected and verified. The oxygen probe is calibrated monthly using an infrared analyzer. Weekly checks of the carbon probe accuracy and the flow settings is accomplished using shim stock processed through the typical austenitize and quench cycle. The proper CO and CO2 concentration is also verified whenever an operator suspects that the operating conditions are not accurate, or when the shim stock shows that the carbon potential is incorrect. While errors in the probe limit the real accuracy of the system, carbon control to 0.50±0.01%C is not uncommon.
During loading of the furnace, the atmosphere is diluted with infiltrated air, and typically, the carbon probe would call for natural gas additions to maintain the carbon potential. This would be computed on the fixed basis of 20% CO. This influx of natural gas yields a carbon spike inside the furnace as the atmosphere and temperature recovers. This introduces a risk of carburization of the part. To avoid this problem, a modification of the typical atmosphere control scheme is necessary. Typically, the reset on the atmosphere controller is inhibited whenever the door is opened. This prevents controller “wind-up” and minimizes the overshoot on the atmosphere. Atimer can be used to start the controller until the atmosphere recovers to approximately 20% CO. In addition, all gas additions (air or natural gas) are delayed until the atmosphere recovers. This is usually accomplished using a time delay in-line with the atmosphere addition valves. Proportional gas addition valves are used to deliver precise gas additions, instead of less expensive and less precise “On-Off” type gas additions. Use of stopoff compounds or copper plating is often used as insurance to prevent decarburization or carburization.
Vacuum oil quenching offers various environmental and economic benefits over traditional hardening techniques in controlled atmosphere furnaces. Because vacuum furnaces are inherently leak tight, control of surface chemistry is precise and the decarburization and high-temperature oxidation problems often associated with traditional controlled-atmosphere heat treating can be minimized. This technique also allows producers of aircraft landing gear to finish machine critical surfaces on these components prior to heat treating. This in turn, reduces final machining costs when the part is in the hardened condition. As a result, many landing gear heat treaters have adopted vacuum oil quenching of landing gear components.

Control of residual stresses and distortion is very critical. Residual stresses, if tensile, can cause premature fatigue cracking. Distortion is difficult and expensive because of the size of the parts, and the heat treated strength of the material. It also can introduce new residual stress fields that can influence fatigue properties. The choice of the proper quenching practice, quenchant, and racking are critical to minimize distortion and residual stresses.Though the use of high pressure gas quenching for hardening certain alloys that were previously oil quenched has been gaining popularity, vacuum oil quench furnaces have a significant role to play in the heat treating field. Gas quenching is limited to the hardening of fairly thin sections of a relatively small number of alloys, so vacuum oil quench furnaces offer an important advantage in a large number of heat treating applications. While gas pressure quenching of landing gear components is being done for smaller components (axles), the application is limited due to the necessary section thicknesses of large landing gear components.
Because the section thicknesses are large, it is often necessary use fast- to-medium-fast oil quenchants, to achieve the through-hardened microstructure requirements. Large variation in hardness from surface to core is not permitted. This is one reason that extreme hardenability alloys such as 300M are used. High quality quench oils are used to achieve uniform quench performance and to achieve load after load consistency. Proactive maintenance programs to control oxidation and minimize staining and to achieve consistent and quenching uniformity are required. Typical times and temperatures are shown in Table 2.
Racking is critical. Care has to be taken to properly balance the load because of the weight. In addition, care has to be taken to properly spread out the load for uniform heat transfer along the length of the parts. Local pockets of heat from the supporting center post are often a cause of longitudinal distortion of several millimeters (up to 12 or so) on a part length of 12 ft (3.5 m). Atypical load for heat treatment is shown in Fig. 2.
Conclusions
The heat treatment of landing gear is a complex operation that requires precise control of the variables of time, temperature and carbon control. Understanding the interaction of quenching, racking, and distortion contributes to reduced distortion and residual stress. The high quality heat treatment of landing gear contributes to our flying safety and excellent safety record.